
A leaf of broad-leaved dock (Rumex obtusifolius), which has been perforated by leaf beetles (Chrysomelidae) and their larvae. Further clutches of eggs are on the leaf lamina. In the right margin of the picture, two spots with red edges (which show the accumulation of anthocyanins) indicate a defence response, probably due to a secondary infection by microbial pathogens. (Photo: E. Beck)
Plants are constantly under attack. They are surrounded by a wide range of potential enemies—organisms that strive to exploit them as substrates. Spores of fungi and oomycetes land on plant leaves all the time and germinate when conditions are right. Insects seek to make the nearest plant their next meal. The soil abounds with bacteria and nematodes, which roots are exposed to. In light of this, plant disease is amazingly rare in wild populations. If it occurs at all, usually only a few plant individuals are affected and the extent of damage is limited to parts of a leaf or a few leaves at most. Most plants remain healthy because they possess an array of preformed (constitutive) and inducible defence mechanisms that restrict the success of potential pathogens or herbivores. Having said this, there are nonetheless instances in which herbivores or pathogens cause devastating damage to one or several species across large areas. One current example is the mountain pine beetle outbreak, destroying large areas of pine forest in British Columbia, Canada. Another one is sudden oak death, a newly discovered disease caused by the oomycete Phytophthora ramorum. Wide areas of California and Oregon in the USA have been affected by the pathogen, which apparently was recently introduced and can colonise not only oaks but approximately 40 other species as well.
As we will see, there are—despite the fundamentally different strategies of, for example, phytopathogenic fungi and chewing insects—many similarities between the strategies plants use to fend off microbial pathogens or herbivores. Both defences can be divided into mechanical/physical and chemical strategies. Both involve recognition events and signalling cascades that result in the induction of defence mechanisms upon the perception of a potential foe or the damage caused by it. There are even common motifs when comparing plant and animal (including human) defence systems. It has in recent years become increasingly clear that they share an innate immunity based on the ability to detect the presence of cells that are foreign to the organism.
The observation and analysis of plant disease are among the oldest questions in biology. Unlike wild plants in their natural habitats, cultivated crops are far more likely to suffer disease (for reasons that will be considered in Sect. 8.2). Because of the yield losses caused by pathogens and herbivores, the ancient Roman writer Columella examined plant disease. Since the nineteenth century, phytopathology has been an active scientific discipline motivated by the goal of reducing susceptibility of cultivated plants to pathogens.
Moreover, plant–pathogen relations serve as an excellent model for the interaction between organisms and their co-evolution. Biotic interactions represent a major driver of biodiversity, and the “warfare” between plant hosts and their potential pathogens explains why (Sect. 8.2).
This chapter first describes the interaction of plants with predominantly microbial and fungal pathogens that can cause disease in plants. We will look at the types of pathogens and their weapons (i.e. pathogenicity determinants), at preformed plant defences and, more prominently, at the inducible plant immune system. The latter encompasses mechanisms involved in the recognition of potential pathogens or their activities, the ensuing activation of defence responses and the apparent co-evolution between hosts and pathogens. The discussion of defence against herbivores (often referred to as plant pests) will be focused on insect herbivores. Finally, the chapter covers damage caused by parasitic plants and the hostile interaction between plants (i.e. allelopathy). Parasitic plants and allelopathic interactions are not nearly as intensively investigated as the plant immune system and herbivore defence. Thus, those parts will present much less molecular insight.
8.1 Plant Disease Caused by Pathogens
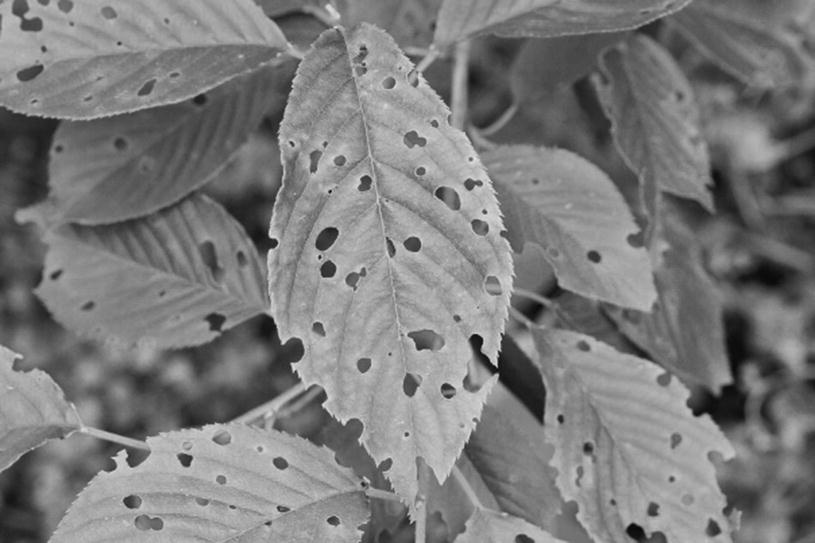
Symptoms of disease caused by plant pathogens. “Cherry spot hole disease” is a generic term used for bacterial leaf spot caused by the bacterium Xanthomonas pruni and cherry leaf spot caused by the fungus Blumeriella jaapii
8.1.1 Types of Pathogens: Viruses, Bacteria, Fungi, Oomycetes and Nematodes
The specificity of plant pathogens can vary widely. Often the host range is restricted to only one species. This applies, in particular, to biotrophic pathogens. However, there are also examples of pathogens infecting multiple hosts. Several necrotrophic fungi (e.g. Botrytis species) cause disease in hundreds of plant species.
Viruses basically consist of a protein coat enclosing nucleic acids (DNA or RNA) that carry the information for the synthesis of only a handful of proteins. For their replication they have to force a host cell to produce these proteins, which then assemble and give rise to new virus particles. Unlike viruses infecting bacterial or animal cells, plant viruses do not have to destroy their host cell in order to release newly formed virus particles, which then attack neighbouring cells. Instead, they move through plasmodesmata from cell to cell and spread systemically via the phloem. Initial infection of a host plant mostly occurs through inoculation of the phloem via virus-carrying phloem-feeding insects such as aphids. Typical symptoms of virus-infected plants are yellowing or a mosaic-like colouration of leaves, stunted growth and sometimes the death of a plant.
Microbial pathogens typically infect only specific tissues or organs. Phytopathogenic bacteria, for example, can cause root rot, leaf chlorosis or stem dieback, depending on the pathogen and its infection strategy. Bacterial pathogens usually enter a plant through wounds or the stomata and multiply in the apoplast. Only a minute fraction of bacterial species have evolved the ability to infect plants. No more than a few hundred species have been described as pathogens of crop plants. Most disease-causing bacteria are gram positive and belong to a limited number of clades (e.g. Xanthomonas, Pseudomonas, Erwinia and Dickeya).
Economically the most important groups of plant pathogens are the oomycetes and fungi. Oomycetes were for a long time regarded as fungi, but phylogenetically they belong to the Chromalveolata and are thus very distant from fungi. Arguably the most famous plant pathogen is an oomycete. Phytophthora infestans is the causal agent of potato blight and was responsible for the Irish famine in the mid-nineteenth century, which left millions of people dead and triggered a mass emigration from Ireland to North America. Downy mildews are oomycetes too.
Among the possibly millions of fungal species living in association with plants, only a small fraction cause disease (Crous et al. 2015). Nonetheless, no other group of pathogens accounts for more yield loss than fungi. Important genera with phytopathogenic species include Botrytis, Alternaria, Colletotrichum, Fusarium and Puccinia.
A few taxa of nematodes are plant pathogens. Presumably because they almost exclusively attack the root system, they are less well studied. Phytopathogenic nematodes are obligate biotrophs—that is, they are absolutely dependent on a plant host for existence. Endo- and ectoparasites are differentiated according to whether they penetrate root tissue or not, respectively. Most of the considerable nematode damage to crop plants is due to infection by cyst and root-knot nematodes.
8.1.2 Pathogenicity Mechanisms
Pathogens have to gain access to the plant’s resources and be able to grow and reproduce rapidly within plant tissues. Both processes require effective suppression of the plant’s immune system. Invasion of plants is achieved through widely different strategies depending on the type of pathogen. As mentioned, viruses are transmitted by insect vectors. The same applies to some phytopathogenic bacteria such as phytoplasma. Most bacteria do not have the ability to actively penetrate plant surfaces. However, after entry through wounds or stomata into the apoplast they can degrade plant cell walls via the secretion of a suite of enzymes such as cellulases and pectinases. Cell wall degradation causes disease symptoms such as softening of tissues. For some Erwinia species it is known that the bacteria “wait” to secrete these enzymes only when a sufficiently large number of bacterial cells are present at the infection site to overwhelm the plant. Earlier release would only alert the plant immune system (as is discussed in Sect. 8.2.2). The “waiting” is mediated by quorum-sensing mechanisms, which bacteria use to monitor the density of a population.

Interaction between the avirulence gene avrBs3 and the resistance gene Bs3. The bacterial pathogen Xanthomonas attacks tomato and pepper cells by injecting effector proteins through the type III secretion system. One of the effectors is avrBs3. It has features of a eukaryotic transcription factor—for example, nuclear localisation signals (NLSs). After entry into the nucleus it activates genes (UPA genes, upregulated by AvrBs3) whose activity causes disease. The matching tomato resistance gene, Bs3, which is present in some tomato cultivars, carries similar promoter elements (UPA box). However, Bs3 activation triggers a cell death programme. The cell undergoes the hypersensitive response, and Xanthomonas cannot spread further. (Modified from Kay and Bonas (2009))
Like many effectors, toxins appear to often function as suppressors of plant defences. This is illustrated by coronatine, a molecule synthesised by Pseudomonas syringae. Coronatine mimics the phytohormone jasmonate and interacts with the jasmonate receptor COI1. Activation of the jasmonate pathway reverses the closure of stomata, which is an integral part of the plant’s defence response (Melotto et al. 2006).
Box 8.1: Agrobacterium tumefaciens

Infection of a plant cell by Agrobacterium tumefaciens. (Buchanan et al. 2015)
When the molecular mechanisms underlying the A. tumefaciens–mediated gene transfer were elucidated in the 1980s, it was immediately realised that it could be utilised to genetically transform plants with other foreign DNA of interest (Caplan et al. 1983). The T-DNA localised between the so-called right border and left border sequences on the tumour-inducing plasmid (Ti plasmid) can be replaced by any DNA, using standard recombinant DNA techniques. Transformed plant cells do not give rise to tumours anymore but instead express genes encoded by the foreign DNA. A typical plant transformation employing A. tumefaciens encompasses the co-cultivation of A. tumefaciens cells carrying a modified Ti plasmid with wounded plant tissue (e.g. leaf discs), selection of transformed plant cells—for example, based on co-transformed antibiotic resistance—and regeneration of a whole plant, using tissue culture techniques.

Compatible a and incompatible b interactions during infection by a fungus. a A germinating spore (S) develops a germ tube from which an appressorium (A) is formed. Mechanical pressure building up in the appressorium, together with the secretion of cell wall–degrading enzymes, allows entry of a primary hypha (P) through the cuticle (C) and cell wall (CW). Later, secondary hyphae (H) grow into the host cell. b Defence against a fungal infection in a resistant plant. Pathogen recognition triggers rapid wall thickening under the appressorium. As a result the fungus is unable to penetrate the outer wall of the epidermal cell. (After Conrath and Kauss (2000))
Necrotrophic fungi do not build haustoria. Instead they overpower a plant with non-specific or host-specific toxins and with cell wall–degrading enzymes. The plant cells die and the fungi utilise the dead organic material. An intensively studied potent toxin is fusicoccin, a diterpene synthesised by Fusarium species. It causes rapid wilting of exposed plants by constitutively activating the plasma membrane H+-ATPase via the modulation of 14-3-3 proteins. In guard cells this activation prevents the closure of stomata because the cells remain hyperpolarised (Chap. 6, Sect. 6.3).
Like bacteria, fungi and oomycetes produce effectors to suppress the plant defence response and to disturb plant development or metabolism. The phytohormone gibberellin was discovered as a molecule the fungus Gibberella fujikuroi uses to trick rice seedlings into abnormal growth. This increases their risk of lodging and thereby falling victim to the fungus. Ustilago maydis, a biotrophic pathogen of maize (causing maize smut, characterised by large tumours), secretes more than 100 effector proteins during its attack. One of them is the enzyme chorismate mutase. Following uptake into plant cells it modulates the phenylpropanoid pathway in a way that synthesis of the defence hormone salicylic acid (Sect. 8.2.3) is disturbed (Djamei et al. 2011).
Nematodes penetrate plant cell walls with feeding stylets. Movement through root tissue is aided by the release of cell wall–degrading enzymes (Davis and Mitchum 2005). Inside plant cells, feeding tubes are formed that extract nutrients from the host. Molecules secreted by the nematode trigger dramatic changes in the regulation and metabolism of root cells, which eventually give rise to giant cells or symplastically connected cells that form syncytial feeding structures for the nematode.
8.2 Plant Defences Against Microbial Pathogens and Viruses
The interaction between a plant and a pathogen can have two principal outcomes. When no disease is caused, the interaction is incompatible. The pathogen is not able to differentiate and reproduce on the plant, because preformed and inducible defences prevent this. The plant is resistant. Successful colonisation, on the other hand, is referred to as compatible interaction. Pathogen propagation occurs at the expense of the host plant. Plant growth is slowed, tissues are damaged and a disease arises. The plant is susceptible, the pathogen virulent.
- 1.
A pathogen cannot develop on a plant, because of efficient preformed defences.
- 2.
None of the races (genotypes) of a pathogen is able to propagate on a particular plant species, because inducible defences restrict the invasion by the pathogen. This is called non-host resistance. The pathogens are non-adapted. Most pathogens are non-adapted to most plants. P. infestans can devastate potatoes, and barley powdery mildew can colonise barley leaves. Both are harmless to Arabidopsis thaliana or most other species, however, because they lack the specific means to overcome the defences of these plants.
- 3.
The resistance is not species wide; it is restricted to certain genotypes (or cultivars in the agricultural context) of a plant species. Some genotypes (races) of a pathogen can propagate on a particular host genotype because they possess effectors that help overcome the plant’s defences. Resistance to other races of a pathogen is based on the recognition of effectors and the induction of the hypersensitive reaction. The pathogen is avirulent because its effectors trigger rapid plant immune responses. The third scenario has long been recognised and described by the gene-for-gene hypothesis (Flor 1971). Genes encoding effectors (traditionally termed avirulence genes because their presence can induce defence in some host genotypes) interact with plant resistance genes (R genes). These encode proteins that recognise effector presence and activate cell death.

Types of plant immunity. (Modified from Nürnberger et al. (2004))
8.2.1 Preformed Defences Against Bacteria, Fungi and Oomycetes
Features of a plant’s morphology and anatomy serve as a first line of defence against invasion by pathogens. The cuticle and cell walls form mechanical barriers against pathogen ingress. Many potential pathogens never gain access to plant cells and their resources, because they lack the means to overcome these barriers.
A characteristic of plant biology is the synthesis of thousands of secondary metabolites—that is, compounds that, in contrast to primary metabolites, are not found in every plant cell and species. Instead, different plants produce different spectra of secondary metabolites, which nonetheless serve important functions in the interaction of a plant with its environment (Bednarek and Osbourn 2009). Because “secondary” might be misunderstood as meaning “of lesser importance”, “specialised metabolites” was proposed as an alternative and perhaps more meaningful term (Pichersky et al. 2006). Nonetheless, throughout this chapter the more common term “secondary metabolites” is used.
Another sensible distinction between primary and secondary metabolites is this: primary metabolites are involved in nutrition and essential metabolic processes; secondary metabolites are involved in the interaction of a plant with its environment (Buchanan et al. 2015). A prominent role is preformed chemical defence against pathogens. Plants produce a rich cocktail of compounds with antimicrobial activity, which are mostly stored in vacuoles or in specialised cells such as trichomes. Sometimes they are referred to as phytoanticipins (Fig. 8.6) and are distinguished from phytoalexins, which are synthesised de novo upon pathogen attack (Sect. 8.2.2).

Phytoanticipins. Saponins such as avenacin A-1 are found in Avena sativa but also in a wide range of other plant species. Glucosinolates are typical of Brassicaceae and other families in the Capparales
A rare alternative to chemical defence is so-called elemental defence. Metal hyperaccumulating plants apparently fend off pathogens and herbivores by accumulating metals such as Zn or Cd to toxic levels in their leaves (Chap. 7, Sect. 7.5.2).
8.2.2 Inducible Local Defences
Notwithstanding the presence of preformed defences, the outcome of an interaction between a plant and a potential pathogen (scenarios 2–4 in Fig. 8.5) is largely determined by the presence or absence and the activities of specific molecules on the host and pathogen sides. The major components are (1) molecules characteristic of potential pathogens (traditionally termed elicitors) and (2) plant receptors that detect such molecules, as well as (3) pathogen effectors (see above), and (4) plant proteins, encoded by resistance genes, which recognise the presence or activity of these effectors.
A characteristic feature of the plant immune system is cell autonomy. Practically every plant cell is capable of mounting defence responses. Thus, many interactions remain highly localised; this is apparent, for instance, as tiny necrotic lesions on leaves, stemming from cell death.
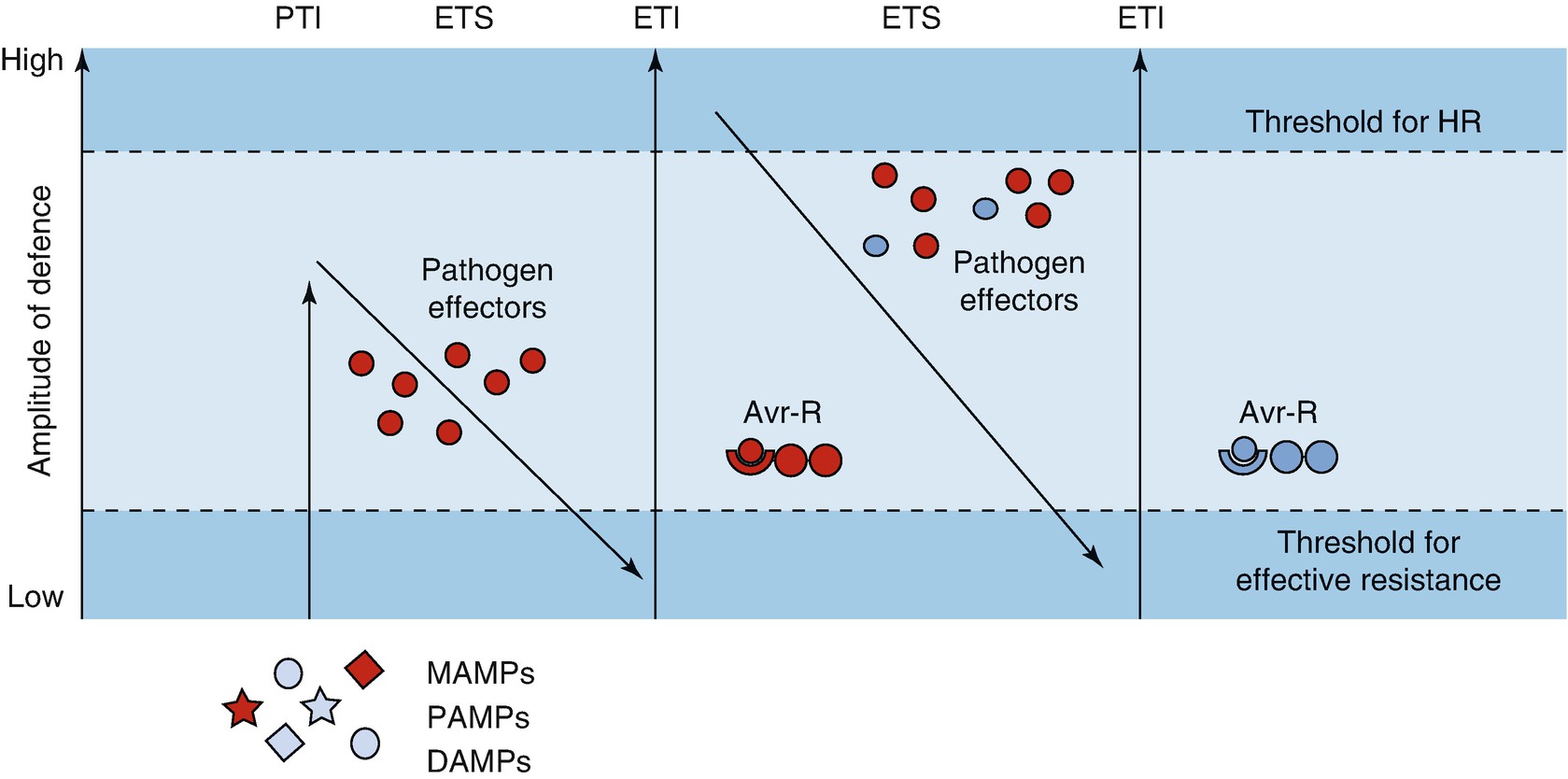
Zig-zag scheme describing plant immunity. Two amplitudes of defence are activated by the recognition of two different types of molecules. The first line of defence depends on the perception of molecules typical of potential pathogens or their activities (microbe-associated molecular patterns (MAMPs), pathogen-associated molecular patterns (PAMPs) and danger-associated molecular patterns (DAMPs)) and is sufficient to prevent colonisation in most cases. The second line of defence recognises effector molecules or the activity of effector molecules produced by specialised pathogens trying to overcome host defences. Activation triggers the hypersensitive response (HR). PTI PAMP-triggered immunity, ETS Effector-triggered susceptibility, ETI Effector-triggered immunity. For more details, see the text. (Modified from Jones and Dangl (2006))
The second, more intensive layer of defence becomes activated when a plant cell recognises an enemy equipped with effectors that could potentially overcome the plant’s defence. The responses are stronger and faster, and can eventually lead to the hypersensitive reaction—that is, localised cell death (scenario 4 in Fig. 8.5). Inability to respond to the effectors results in susceptibility (scenario 3 in Fig. 8.5).
In cases where preformed defences are not sufficient to completely prevent the activity of a potential pathogen, the first layer of inducible defence, the innate immunity, becomes active. Plant cells respond to the presence of a potential foe with the induction of various defence mechanisms. Antimicrobial compounds (phytoalexins) are synthesised, cell walls are locally reinforced and defence proteins such as enzymes (e.g. chitinases) to attack microbial cell walls are produced.

A pathogen attack triggers highly localised defence responses. Phytoalexin accumulation (reddish inclusion bodies (IB)) and cell wall modification (arrows) are visible at an infection site on a Sorghum leaf. (Snyder and Nicholson 1990)
Likewise, cell walls are strengthened locally—for instance, right underneath an attempted penetration site of a biotrophic fungus (Fig. 8.4b). Small papillae are formed by deposition of callose (a β-1,3-glucan) and lignin. Cross-linking of extracellular proteins with the cell wall matrix further reinforces the mechanical barrier to fungal ingress.
A third element of the defence response is the synthesis of many pathogenesis-related proteins (PR proteins) which, contrary to what the name suggests, are in fact proteins thought to have antimicrobial activity. As for a range of other stress-related proteins in plants (see dehydrins in Chap. 6), their actual biochemical function is poorly understood. Exceptions are chitinases and glucanases, which attack fungal cells walls.

Pathogen-associated molecular pattern (PAMP)–triggered immunity (PTI) and effector-triggered immunity (ETI). Perception of PAMPs (or microbe-associated molecular patterns (MAMPs) or danger-associated molecular patterns (DAMPs)) by pattern recognition receptors (PRRs) and their co-receptors (e.g. BAK1) triggers PTI responses. Specialised bacterial, fungal or oomycete pathogens release effector molecules to suppress PTI responses. Effectors or their activities are recognised by resistance gene products, typically nucleotide-binding domain leucine-rich repeat domain (NB-LRR) proteins. This event triggers ETI responses such as programmed cell death. For the sake of clarity, the plant cell wall is not shown. (Modified from Dodds and Rathjen (2010))
The number of PRRs per plant species is estimated at around 100–200. Together they constitute an effective surveillance system that enables plant cells to sense the extracellular presence of many different potential microbial pathogens. The innate immunity based on this surveillance system is mechanistically very similar to animal innate immunity. However, plant cells express far more PRRs than animal cells. This is regarded as one way to compensate for the absence of an adaptive immune system in plants (Dodds and Rathjen 2010).

Signal transduction in pathogen-associated molecular pattern (PAMP)–triggered immunity (PTI) and effector-triggered immunity (ETI). PAMP recognition triggers mitogen-activated protein (MAP) kinase phosphorylation cascades, Ca2+ spikes, modulation of ion fluxes (plasma membrane depolarisation) and an oxidative burst (reactive oxygen species (ROS) formation). Defence genes are activated and the cell wall is reinforced by callose deposition. Signalling downstream from effector recognition (shown on the right side in the scheme) is poorly understood. (Modified from Buchanan et al. (2015))
Transduction of the signal from activated PRRs to ROS-producing enzymes and to transcription factors proceeds via multiple phosphorylation steps. PRRs themselves are receptor kinases. Upon ligand binding they interact with co-receptors and become activated (Fig. 8.10). Ca2+ spikes and the activation of mitogen-activated protein kinase cascades (MAP kinase cascades) eventually modulate a complex network of transcriptional regulators that ensures an adequate response—that is, a reprogramming of the cellular metabolism, which is adjusted to the severity of the pathogen threat (Tsuda and Somssich 2015). Like all plant stress responses, PTI represents a balancing act to optimise resource allocation between defence and growth.
The second layer of defence is activated when pathogen effectors are recognised. At this level of immunity most of the co-evolutionary dynamics take place because unlike MAMPs/PAMPs/DAMPs, effectors are highly variable and also dispensable—that is, not essential for normal cell functioning (Dodds and Rathjen 2010). Therefore, effector recognition requires large sets of resistance genes (R genes), which are plant species specific and even genotype specific, while many PRRs are conserved across plant families because they perceive PAMPs, which are widely conserved as well. R gene products mediate the effector perception and the resulting effector-triggered immunity (ETI; see zig-zag scheme in Fig. 8.7).

Activity of a fungal effector. The fungal effector Ecp6 is produced by the fungal plant pathogen Cladosporium fulvum. It suppresses the recognition of danger-associated molecular patterns (DAMPs) by binding to chitin fragments. An oxidative burst of Nicotiana benthamiana cells, visualised as the chemiluminescence of oxidised luminol, upon treatment with fragments of chitin (GlcNAc)6 is shown. (de Jonge et al. 2010)
Upon recognition of an effector, a plant cell mounts a strong and rapid response that confers ETI. The most extreme manifestation of this response is the hypersensitive reaction, meaning programmed cell death, which effectively blocks further spread of an invading pathogen.
ETI is dependent on R genes. R genes can confer resistance to fungi, oomycetes, bacteria, nematodes and even viruses. They have been used extensively in crop breeding to achieve resistance against commercially important pathogens. Their molecular nature, however, was not known until 1995. Most R genes encode proteins that share certain structural domains—namely, leucine-rich repeats (LRRs), often involved in intermolecular interactions—and nucleotide-binding (NB) domains. There are, however, intriguing exceptions. The R gene Bs3 in pepper “outsmarts” pathogens carrying the effector avrBs3 (Fig. 8.2). When activated, Bs3 triggers a hypersensitive reaction (Römer et al. 2007). Bs3 is activated by avrBs3 binding because the Bs3 promoter carries the same cis element that is targeted by avrBs3 to cause disease. In other words, by attacking a plant cell with Bs3 in its genome, Xanthomonas campestris terminates its own infection attempt.

The “guard hypothesis”. Resistance proteins (R proteins) recognise either effectors (encoded by avr genes) or the modification of critical virulence targets by effectors. Both scenarios trigger a hypersensitive response and confer resistance; the interaction is incompatible. Successful attack on a virulence target compromises immunity and contributes to pathogen propagation (compatible interaction)
Another reason why plants are not overwhelmed in the “arms race” with microbial pathogens is the diversity of R genes, which is driven by the continuous emergence of pathogen effectors. Plant genomes carry large numbers of R genes, many of them organised in clusters facilitating recombination events. R genes are consistently the most polymorphic class of genes in plant genomes. Thus, within a given plant population, hundreds or thousands of R gene variants exist that can sense effector-dependent pathogen attacks. Genetic diversity within a population reduces the vulnerability to pathogens.
The second function of R gene products, besides direct or indirect recognition of pathogen effectors (i.e. signalling to activate cell death programmes (Figs. 8.9 and 8.10)), is molecularly poorly understood.
Box 8.2: Ug99 Stem Rust on Wheat

Ug99 resistance conferred by transforming wheat with the resistance gene Sr35. Infection symptoms on seedlings of transgenic lines segregated for the resistance transgene. Plants carrying the transgene (+) were Ug99 resistant (R), plants without the transgene (−) were susceptible (S). (Saintenac et al. 2013)
8.2.3 Inducible Systemic Resistance
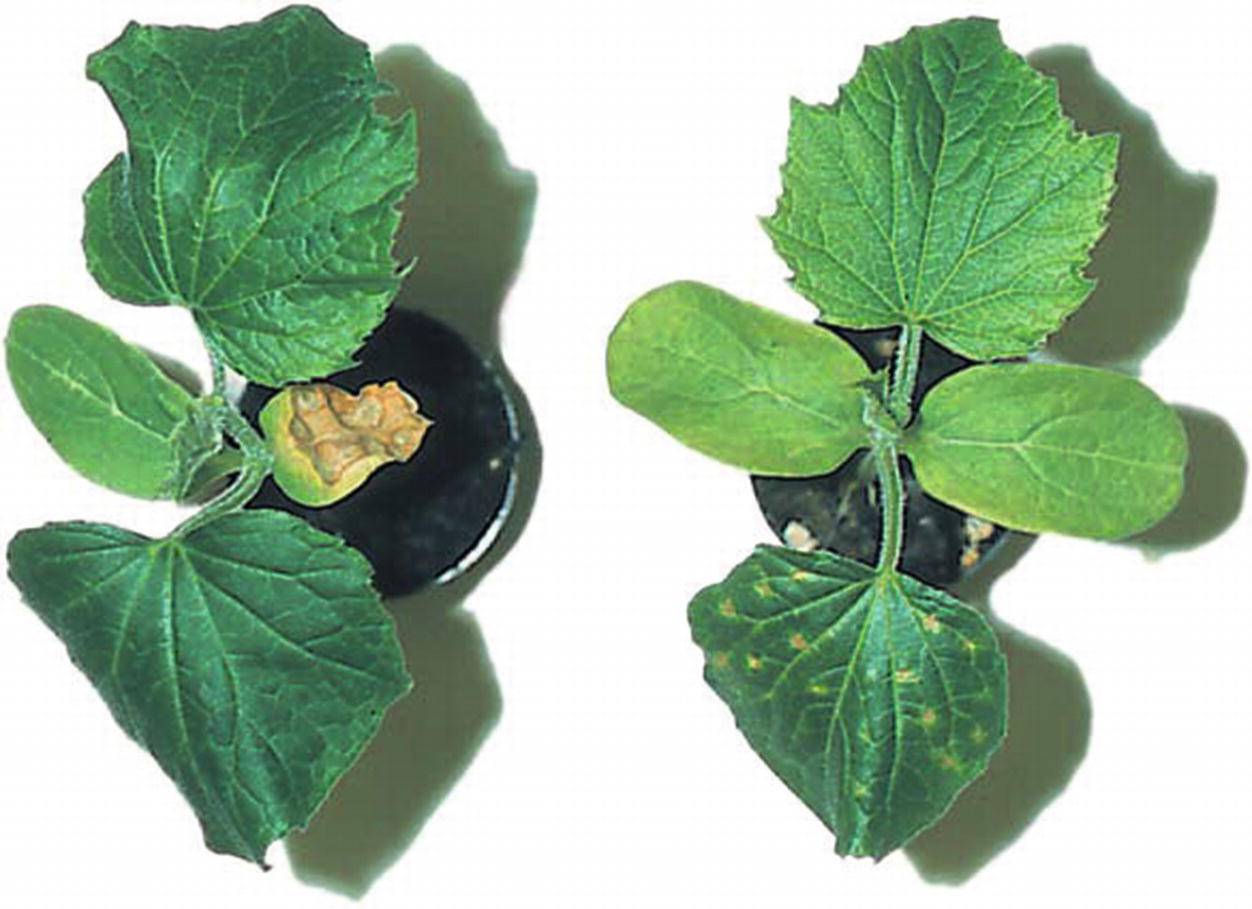
Systemic acquired resistance of cucumber plants to the anthracnose fungus Colletotrichum lagenarium. One cotyledon of the plant on the left was infected with spores of the fungus. It is suffering severely from necrosis. The plant on the right was left untreated. One week later, 20 drops of a spore suspension were placed on a main leaf of both plants. The leaf of the plant on the left remained fully healthy, as it had developed systemic resistance. The leaf of the plant on the right, which experienced no “immunisation”, exhibited necrotic areas at the positions of every drop of spore suspension. (Conrath and Kauss 2000)

Systemic induction of pathogenesis-related (PR) genes. Treatment of Arabidopsis thaliana leaves with the defence hormone salicylic acid (SA) or inoculation with Pseudomonas syringae strongly activates PR gene (PR-1, PR-2, PR-5) expression. Expression of PR genes was analysed by northern blot hybridisations. C control, Pst Pseudomonas syringae. (Uknes et al. 1992)

Systemic acquired resistance. MeSA Methylsalicylate. (Buchanan et al. 2015)
Arrival of the signals in the systemic tissue again results in an increase in the salicylic acid concentration, albeit smaller than that in the local tissue. Salicylic acid is perceived by different receptors locally and systemically. In cells under direct pathogen attack, salicylic acid positively regulates cell death, while in systemic tissue it suppresses cell death and sets signalling cascades in motion that result in PR gene activation and SAR.
8.2.4 Defence Against Viruses via Gene Silencing
Defence against the systemic spread of viruses is conferred not only by the R gene–mediated hypersensitive response or by inducible systemic resistance; in fact, the main immune system against viruses in plants is based on gene silencing. For decades it has been observed in the field and under laboratory conditions that virus-infected plants develop resistance against a second infection by the same virus or a closely related virus. Weeks after an infection the newly developed leaves remain symptom-free even when inoculated with virus particles (Soosaar et al. 2005). This phenomenon, called “recovery”, resembles the immunisation of humans or other mammals through treatment with an inactivated virus or a harmless related virus. We now know that a gene-silencing mechanism is behind the recovery from virus infection (Baulcombe 2004). This silencing targets virus-derived double-stranded RNA (dsRNA), which also explains why resistance covers not only the originally infecting virus but also viruses with a genome that is similar in sequence.

Virus-induced gene silencing. The presence of double-stranded RNA (dsRNA) during the replication of viral genomes triggers processing by RNase-like plant enzymes (Dicer and Dicer-like (DCL)). The resulting small RNAs are loaded into Argonaute (AGO)-containing RNA-induced silencing complexes (RISCs) to guide translational inhibition and/or slicing of viral RNA. The virus-derived small RNAs have the potential to spread through plasmodesmata to neighbouring cells, thereby activating a systemic antiviral defence (= immunisation). On green background the RNA silencing triggered by RNA viruses is depicted. Brown background and yellow symbols indicate the additional defence against DNA viruses via the modification of their DNA genomes (methylation of DNA or histones) by small RNAs. Red symbols indicate viral suppressors of RNA silencing, produced by viruses to counteract the antiviral defence of the plant. P plasmodesmata. (Incarbone and Dunoyer 2013)

Spreading of gene silencing confers resistance against viruses. Non-transformed Nicotiana benthaminana plants (nt) and plants with strongly reduced expression of the RNA-dependent RNA polymerase RDR6 (RDR6i) were inoculated with potato virus X (PVX). RDR6 is required for the response of cells to the systemic silencing signal. Stunting of RDR6i plants compared with nt and non-inoculated plants (ni) indicates loss of virus resistance. dpi days post infection. (Schwach et al. 2005)
8.3 Herbivory

Insect herbivores. a Feeding tunnels of leaf miners (Photo: copyright obtained from Shutterstock). b Monarch (Danaus plexippus) caterpillar feeding on a leaf of milkweed (Asclepias syriaca). (Photo courtesy of the National Science Foundation, USA)
A second fundamental distinction, in addition to the feeding style, is that between generalist herbivores (which do not show much preference for certain plant species) and specialist herbivores (which seek particular groups of plants as food source). Specialisation is a consequence of the ability these insects have evolved to overcome the defences typical of individual plant families such as the Brassicaceae (Sect. 8.3.1).
In spite of the abundance of herbivorous insects with their need to access the resources provided by photosynthesis, damage on plants is usually limited. Constitutive and inducible defences of plants act as effective barriers to the consumption of plant tissue by herbivores. While the discussion in this chapter focuses on insect herbivores, it should be noted that principally these defence mechanisms effectively restrict damage by vertebrate herbivores as well. Constitutive defences include morphological features such as thorns and trichomes. However, plant herbivore defence is predominantly chemical (Mithöfer and Boland 2012). Low molecular weight compounds and proteins act as feeding deterrents or as toxins with direct inhibitory effects on insect growth and fitness. Molecules of chemical defence either are preformed and stored or are synthesised in response to the presence of feeding insects. Similar to pathogen defence, the inducible defence can be local and systemic.

Layers of herbivore defence
8.3.1 Constitutive Defences
Plants rely heavily on chemical defences against herbivores. As discussed in Sect. 8.2.1, plants produce a plethora of secondary metabolites. More than 200,000 different chemical structures have been identified and presumably many more exist in nature. Per plant species, several thousand of these metabolites can be expected. A major biological role is protection against herbivore attack.

Typical secondary metabolites with herbivore defence function. The steroid digoxin is a cardiac glycoside. It was isolated from the foxglove plant, Digitalis lanata, and is used as a treatment for various heart conditions because of its inhibitory effect on Na+-/K+-ATPases. Amygdalin is a cyanogenic glucoside that releases hydrogen cyanide. The alkaloid nicotine is synthesised in tobacco plants as a feeding deterrent and defence compound
About 20% of all plant species produce alkaloids—N-containing metabolites synthesised from various amino acids. Many alkaloids are known as potent toxins, effective against both arthropods and vertebrates. Modes of toxicity vary. Some alkaloids affect the nervous system. For example, caffeine (an alkaloid of Coffea arabica and many other plant species) can have a paralysing effect on insects by inhibiting phosphodiesterase activity at synapses. Another famous example of a toxic alkaloid with a defence function is nicotine (Fig. 8.21). Nicotine is produced in the roots of Nicotiana tabacum and transported via the xylem to leaf cells, where it is stored in vacuoles. In insects it inhibits abundant postsynaptic receptors, the nicotinic acetylcholine receptors, thereby affecting control over muscle movement. Nicotine can, in addition, act as a deterrent. Using transgenic Nicotiana attenuata plants with blocked nicotine biosynthesis, it was shown that nicotine in floral nectar reduces nectar robbery and at the same time modulates the behaviour of pollinators in a way that increases reproductive success. Hummingbirds feeding on nicotine-containing nectar are discouraged from staying too long at any one flower by the unpleasant taste of nicotine. This results in more cross-pollination of tobacco plants and thereby promotes genetic diversity (Kessler et al. 2008).
Phenolic compounds (or phenolics) comprise a huge and chemically diverse group of metabolites sharing an aromatic ring with at least one hydroxyl group. They are found in all plant groups and have a wide range of functions—for example, as major constituents of plant cell walls (lignin, suberin), as flower colours (anthocyanins) or as ultraviolet protectants (flavones, flavonols). Many phenolics act as toxins or antifeedants. Tannins are synthesised by the majority of plant species. In the leaves of many woody dicots they accumulate to concentrations of up to 10% of dry weight (Barbehenn and Constabel 2011). Two major types are distinguished: hydrolysable tannins are polymers of simple phenolics and sugars and are localised mainly in cell walls; condensed tannins are formed by the polymerisation of flavonoids and are stored in vacuoles. Tannins can protect plants because they act as strong feeding deterrents for invertebrates and vertebrates. Beavers, for instance, prefer low-tannin poplars to high-tannin poplars. The toxic effects of tannins are not understood in molecular detail and are thought to be due either to non-specific interaction with proteins or to pro-oxidant activities that result in oxidative stress in the gut of herbivorous insects. Overall, tannins can serve as an example of the lack of direct evidence as to the exact activity and effects of compounds widely implicated in chemical defence. In the absence of clearly defined genotypes that differ only in the molecules in question, it is difficult to infer the actual consequences of these molecules for the interaction of a plant with its herbivores. Such ecological research greatly benefits from genetic modification, as demonstrated by the nicotine example described above (Kessler et al. 2008).

Community phenotypes of poplar trees. The composition of communities living on and around a Populus angustifolia tree are strongly influenced by the genotype of the poplar individual, as determined in common garden experiments. This is apparent from the high degree of heritability (H2 and H2 c)—that is, the large proportion of phenotypic variance attributable to genetic variance. One of the genetically determined variable traits is the content of hydrolysable tannins. (Whitham et al. 2008)

Plant volatiles enable indirect herbivore defence. Choice experiments with parasitic wasps showed they prefer maize plants with caterpillar damage (Cat) over maize plants with artificial damage (Art) (A). Artificial damage treated with regurgitant (Art + Reg) (B, C) was nearly as attractive as caterpillar damage. Total numbers of flights are given with each bar. Asterisks indicate significant differences. (Turlings et al. 1990)

Attraction of herbivore parasitoids by a volatile defence signal. Arabidopsis thaliana plants transformed with a terpene synthase (TPS10) produce volatile sesquiterpenes normally released by maize plants. Experienced individuals of the parasitic wasp Cotesia marginiventris prefer transgenic A. thaliana plants to wild-type plants. Transgenic TPS10 sesquiterpene–releasing Arabidopsis and wild-type plants were placed in two arms of a six-arm olfactometer a to test the attraction of parasitoid females b. Three groups of parasitoids were tested c: naive wasps, wasps with a previous oviposition experience in a host larva in the presence of transgenic Arabidopsis emitting TPS10 sesquiterpenes, and wasps with a previous oviposition experience in a host larva feeding on maize. **Significant preference (p < 0.01) for the odour of the transgenic A. thaliana. (Schnee et al. 2006)
Direct defence is mediated, for instance, by the feeding deterrence of terpenoids in essential oils or resins, as well as the terpenoid volatiles emitted by some plants (Unsicker et al. 2009).
Steroids are triterpenes and thus are synthesised from isoprene units too. Among them are not only saponins (Fig. 8.6) but also cardenolides, the so-called cardiac glucosides (Sect. 8.2.1). They inhibit Na+-/K+-ATPase and thereby interfere with maintenance of the membrane potential in neurons. Related molecules with a likewise known mode of toxicity are phytoecdysons. They mimic insect hormones such as ecdysone and thereby interfere with developmental processes in insects.

Conjugated defence compounds and release of toxic compounds upon tissue damage. a The cyanogenic glucoside amygdalin occurs in seeds and leaves of many plant species. Upon wounding, the glucosidases amygdalin hydrolase and prunasin hydrolase become active. Following cleavage of the sugar moieties, hydroxynitrilases release hydrogen cyanide. b When cabbage leaves are damaged, the mustard oil glucoside sinigrin is released from the vacuole and comes into contact with a thioglucosidase (myrosinase). Removal of glucose by myrosinase results in a reactive intermediate, which is converted into volatile allyl isothiocyanate (allyl mustard oil). This compound, which has a pungent flavour to humans, is extremely toxic to many insects. For the specialist herbivore Pieris brassicae (the large cabbage white butterfly), the allyl mustard oil is, however, an attractant, which stimulates oviposition by the female butterfly
A second important class of conjugated defence compounds is the glucosinolates—sulphur-containing specialised metabolites typical of the order Capparales. Because Brassicaceae belong to this order and the model species A. thaliana belongs to the Brassicaceae, the mechanisms, evolution and diversity of glucosinolate biosynthesis are molecularly better understood than those of most other defence compounds (Box 8.3). Glucosinolates are sometimes referred to as the “mustard oil bomb”. They are synthesised from various amino acids (e.g. methionine, alanine, phenylalanine, tyrosine and tryptophane) and stored in a glycosylated form in vacuoles. Upon tissue damage, glucosinolates come in contact with myrosinases—thioglucosidases that remove the sugar. Myrosinases are present only in a few scattered cells (Fig. 8.25). The aglycones arising from the sugar cleavage are unstable and spontaneously rearrange into a variety of bioactive compounds, including isothiocyanates and nitriles. Isothiocyanates, in particular, are highly reactive and toxic to insects, mammals and many other herbivores. Volatile isothiocyanates are, in addition, known to attract organisms of the third trophic level (i.e. parasitoids of herbivores) and thus contribute to indirect defence (Hopkins et al. 2009). On the other hand, some herbivores specialising in Brassicaceae have evolved the ability to use isothiocyanates as cues for finding their host plant. These multiple roles of glucosinolates are major drivers of genetic diversity in the biosynthesis machinery (Box 8.3).
Besides the amino acids used for protein biosynthesis, plants produce numerous non-proteinogenic amino acids as important primary metabolites (e.g. S-adenosylmethionine) or as secondary metabolites. Most of the latter are specific to particular plant species. Fabaceae tend to produce more non-common amino acids than other plant families. Among the functions of these metabolites is herbivore defence. The best-documented example is canavanine, an important nitrogen storage compound in the seeds of jack beans and other legume species. Canavanine is an arginine analogue, can be incorporated into proteins in place of arginine and is therefore highly toxic to organisms ranging from bacteria to man (Huang et al. 2011).
Constitutively synthesised defence compounds are found in all plant organs. Concentrations can vary strongly depending on the developmental stage, the environmental conditions (see inducible defences in Sect. 8.3.2) and also the individual genotype (e.g. the tannin example discussed above). A substance particularly rich in defence compounds is latex, an emulsion exuded by about 10% of all plant species when mechanical damage occurs (Agrawal and Konno 2009). Probably the most famous example of a defence compound in latex is morphine in Papaver species. In fact, many of the compound classes discussed above can be present at higher concentrations in latex than in leaves. This is why plant latex is sometimes compared with animal venoms, a complex mixture of low molecular weight and proteinaceous defence molecules, which exert a multitude of effects on a range of species. To date, few of the effects have been resolved molecularly. Mulberry leaves are toxic to insects other than the silk-producing Bombyx mori. Part of the toxicity can be attributed to alkaloids in the latex that resemble sugar molecules and thereby inhibit glucosidases. They are present in high concentrations (>1.5%; about 100-fold higher than in leaves) and are toxic to caterpillars (Konno et al. 2006).
An alternative to chemical defence has evolved in a limited number of plant species: hyperaccumulation of potentially toxic elements in leaves (= elemental defence; Sect. 8.2.1). Selenium hyperaccumulation is the best-documented example; hyperaccumulation of Cd, Zn and Ni may serve similar functions (Cappa and Pilon-Smits 2013).
8.3.2 Inducible Defences Against Herbivores
The previous subchapter provided a glimpse of the huge variety of molecules synthesised by plants that influence the interaction with herbivores, as toxins, deterrents or attractants. While plants produce a complex cocktail of secondary metabolites constitutively, there is, in addition, the induced chemical defence, which comprises not only metabolites but also defence proteins and changes in the storage sites of carbohydrates to minimise the consequences of tissue loss. The advantage of induced defences is that the expenditure of resources is restricted to periods when defence is actually needed, thereby reducing the costs of defence, in favour of growth and reproduction. On the other hand, they have to be activated quickly in order to be effective.

The systemic wound response. MeJA Methyljasmonate. (Buchanan et al. 2015)
Induced herbivore defences—and, in particular, the systemic responses—add another layer of complexity to interactions within the communities of arthropods, etc., around a plant. Inducible defences connect organisms through space and time. Activities of root-feeding herbivores, for example, influence the chemical composition of above-ground tissues and, with that, the behaviour of organisms responsive to plant volatiles, etc. Similarly, herbivory early in the growing season has long-lasting systemic effects on a plant’s biochemistry and, again, significantly impacts the interacting organisms later in the year.

Indirect herbivore defence via a multitude of tritrophic interactions. Biological effects and interactions of volatile organic compounds (VOCs) and extrafloral nectar (EFN). (1) Feeding by a herbivore. (2) Jasmonic acid (JA) synthesis. (3) Inducible release of VOCs and EFN from wounded and intact leaves. (4) Some VOCs can induce indirect defences systemically—that is, in as-yet-unattacked leaves. (5) and (6) VOCs attract parasitic wasps that parasitise herbivores. Attraction of predatory mites (7). Wasps and mites also feed on EFN, as do ants (8). (9) Both con- and heterospecific herbivores can be attracted by VOCs (9), as well as repelled (10). (11) VOCs can also be perceived by other plants of the same species or a different species. (12) Underground feeding by, for example, beetle larvae can induce release of VOCs from roots (13) and elicit a systemic signal triggering EFN production or VOC release from above-ground organs (14). MeSA Methyl salicylate, TMTT 4,8,12,-Trimethyl-1,3,7,11,-tridecatetraene. (Heil 2008)
Most of the defence molecules described above are synthesised throughout the life cycle of a plant. Upon herbivore attack, production of some can be strongly up-regulated, so they are part of both the constitutive and the inducible defences. As a consequence, the attacked plant becomes more toxic and unpalatable. A further step towards this state is the synthesis of proteins that can affect the fitness and growth of herbivores. What is well documented is the production of protease inhibitors that interfere with an insect’s digestive system. A range of different proteins complements the plant defence arsenal. Oxidative enzymes such as lipoxygenases and polyphenol oxidases have the potential to cause damage to insect tissues. Lectins and other carbohydrate-binding proteins can inhibit sugar-containing molecules in the insect gut. Cysteine proteases damage the peritrophic membrane that protects the gut epithelium. Arginine and threonine deaminases degrade essential amino acids. A feature that many of the plant defence proteins have in common is that they are comparatively resistant to gut proteases. This enables their biochemical identification via selective enrichment during gut passage (Howe and Jander 2008).
Indirect defences can be induced as well (Fig. 8.27). Changes in the blend of volatiles released by a plant upon herbivore feeding are either due to the processing of stored conjugated specialised metabolites (e.g. glucosinolates; Fig. 8.25) or attributable to newly synthesised volatile compounds. A different type of indirect defence relies on rewarding the enemies of herbivores. Secretion of extrafloral nectar attracts non-herbivorous ants and other predators of herbivores (Heil 2008) (Fig. 8.27). The exact chemical composition of such nectars is not known. In an analogy to floral nectaries the presence of sugars such as glucose and fructose, as well as secretion mediated by sugar transporters, can be assumed.
8.3.2.1 Recognition of Herbivore Attack
Rapid induction of direct and indirect herbivore defences is of course dependent on reliable cues perceived by the plant. Insect feeding causes mechanical damage, and plants indeed respond to this (wounding response). However, detailed comparative studies—for instance, of transcriptome changes—have clearly shown that a plant differentiates between the tissue loss due to mechanical wounding and the tissue loss caused by a feeding insect. The pattern of gene induction and repression is clearly distinct for the two conditions (Howe and Jander 2008). Thus, there are apparently signals associated with the insect’s activity that provide information for the plant. Sources of such signals can be insect saliva, regurgitant (another form of oral secretions produced by lepidopteran larvae), oviposition fluids or faeces. In analogy to the term MAMPs used in the context of pathogen defence, these signals are now referred to as HAMPs (herbivory associated molecular patterns) or insect elicitors. In addition, the more general term DAMPs is used as well, summarising signals arising from the plant because of enemy activities (e.g. mechanical damage). In fact, the concept of DAMP recognition unifies induced plant immunity to pathogens and herbivores.
The first HAMP identified was volicitin, a fatty acid–amino acid conjugate isolated from oral secretions of beet army worm caterpillars (Spodoptera exigua) (Alborn et al. 1997). The conjugate is derived from a plant fatty acid, while the glutamine and the conjugation reaction stem from the insect. Treatment of artificially wounded maize plants with volicitin activates the production of volatiles typical of the herbivore response, while the wounding alone does not lead to production of the same volatile blend. Since the discovery of volicitin, several other HAMPs have been molecularly identified: a range of other fatty acid–amino acid conjugates, breakdown products of plant adenosine triphosphate (ATP) synthases called inceptins and sulphated fatty acids called caeliferins (Acevedo et al. 2015).
HAMP receptors are not known yet. Generally, recognition of HAMPs by plant cells is molecularly less well understood than MAMP recognition. The same is true of the early signal transduction events triggered by HAMP sensing, even though the existing knowledge shows extensive principal similarity to pathogen responses. The same basic modules are implicated: transient cytosolic Ca2+ spikes, membrane potential changes, ROS as signalling molecules, and mitogen-activated protein kinase cascades (Wu and Baldwin 2010).
As in the plant–pathogen interaction, there is evidence that herbivores produce effectors to overcome plant defences, and that plants have evolved the ability to sense such effectors or their activities. Again, the molecular understanding is far less advanced, at least partly because plant–herbivore interactions are less amenable to the type of genetic analysis that led to the gene-for-gene concept. An example of herbivore effectors are ATPases in insect saliva. Mechanical damage of plant cells causes a release of ATP into the extracellular space. This extracellular ATP can serve as a DAMP for the plant, and insect ATPases degrade this potential signal. Thus, a plant receptor for extracellular ATP presumably functions in DAMP recognition (Choi et al. 2014).

Jasmonate biosynthesis is essential for effective herbivore defence. The tomato defenceless mutant (left)—which is deficient in jasmonate biosynthesis—and wild-type plants (right) were exposed to hornworm larvae for 13 days. The right panel shows the larval size after feeding on the mutant (left) and wild-type plants. (Howe et al. 1996)

Jasmonate-triggered herbivore defence. Danger signals derived from attacking herbivores (herbivory associated molecular patterns (HAMPs)) and damaged plant cells (danger-associated molecular patterns (DAMPs)) are recognised by pattern recognition receptors (PRRs) at the cell surface. PRR activation triggers intracellular signalling systems involving mitogen-activated protein (MAP) kinase pathways, Ca2+ spikes and reactive oxygen species (ROS) production. In a largely unknown fashion these signalling events activate jasmonic acid (JA) biosynthesis from its precursor, linolenic acid (LA), in plastids and peroxisomes. JA is the substrate for synthesis of the actual signal JA–isoleucine (JA-Ile) in the cytosol. In the nucleus, JA-Ile promotes JAZ-COI1 interaction and targets JAZ repressor proteins for proteolytic degradation by the ubiquitin-proteasome system (Fig. 8.30), thereby activating the expression of JA-responsive genes and the expression of defence traits. JA signalling is attenuated by catabolism of JA-Ile via ω-oxidation and hydrolysis, synthesis of JAZ repressors that are stable in the presence of JA-Ile, and transcription factors (JAMs) that negatively regulate JA response genes. OPDA 12-oxo-phytodienoic acid, β-ox β-oxidation, JAM JASMONATE-ASSOCIATED MYC2-LIKE, JAZ Jasmonate Associated Zinc-finger domain, 12-carboxy-JA-Ile 12COOH-JA-Ile. (Modified from Campos et al. (2014))

Activation of defence genes upon jasmonic acid–isoleucine (JA-Ile) perception. JAZ proteins (blue bar), supported by co-repressors (CR), bind and repress transcription factors (TF) associated with the promoters of early genes in the JA response. JA-Ile binding to the receptor COI1 promotes interaction of the SC-COI1 E3 ligase complex and the tagging of JAZ for degradation in the proteasome. As a result, early JA response genes can now be transcribed. (Browse 2009)
Herbivore defence responses are analogously switched on in tissues distant from the site of herbivore-inflicted damage. JA or JA conjugates are phloem-mobile signals, which may travel to as yet unaffected tissues. In addition, volatile methyljasmonate serves as an airborne long-distance signal (Fig. 8.26). The JA-dependent activation appears to be universal in the plant kingdom. Besides this central mechanism, there are other known signals and signalling modes. The systemic wound response was discovered and intensively investigated in tomato plants. An early breakthrough was the identification of systemin, a peptide signal derived from a pre-protein through proteolytic processing (Pearce et al. 1991). Systemin is produced in wounded tomato leaves and activates the JA pathway in neighbouring vascular tissues. It can thus be considered as a DAMP released by wounding. Orthologous peptides with comparable activity have never been found, suggesting that systemin and systemin-like peptides are restricted to a small group of plant species. Nonetheless, defence-activating peptides of a different molecular nature have in the meantime been identified, and they appear to be widespread in the plant kingdom (Bartels and Boller 2015).
A systemic wound response dependent on JA as the mobile signal takes a few hours to materialise. An alternative mode of long-distance signalling could be electrical. Indeed, the up-regulation of JA synthesis in distal tissues within minutes of wounding appears to depend on electrical signalling (Mousavi et al. 2013). Both insect feeding and wounding elicit surface potential changes in A. thaliana leaves, which travel through the rosette to distal leaves at an estimated speed of around 6 cm/min. The amplitude of the signal correlates with the strength of the JA response activation in these leaves. Conversely, the injection of current into leaves systemically triggers JA synthesis in the absence of wounding. Ion channels involved in the generation and propagation of the electrical signal are glutamate-receptor-like proteins.
8.3.3 How Plant–Herbivore Interactions Drive Genetic Diversity
The interaction of plants with the plethora of herbivorous animals surrounding them is generally regarded as a major driver of biodiversity. This has in a few cases been possible to demonstrate experimentally. In field experiments with a native plant, Oenothera biennis, it was shown that the suppression of insect herbivores in one half of the plants by insecticide treatment not only suppressed herbivore-inflicted damage but also resulted in rapid evolutionary change, apparent as genetic differentiation between the populations. Over five growing seasons, herbivore defence declined in the protected populations, for instance, because of lower elagitannin levels in fruits (Agrawal et al. 2012). Conversely, competitive ability increased. Such experiments document evolutionary consequences of a trade-off between defence and growth in real time.

Evolution in action: herbivores differentially select glucosinolate chemotypes. A multigeneration selection experiment was performed with an ancestral population consisting of 27 Arabidopsis thaliana accessions differing in the type of methionine-derived glucosinolates (orange: C3 chemotype, green: C4 chemotype). The populations were exposed to one aphid species (Brevicoryne brassicae, Lipaphis erysimi or Myzus persicae) or a combination of them. The pie charts illustrate the changes in the composition of A. thaliana accessions from equal proportions of 27 genotypes to treatment-specific compositions after five generations of selection. The mean genotype frequencies based on six replicate populations are shown. For example, the accession Sap-0, which produces much smaller amounts of glucosinolates than the other accessions, became dominant in the absence of herbivore pressure but was eliminated from the population in the presence of aphids. (Züst et al. 2012)
Box 8.3: The GS-ELONG Locus in Arabidopsis thaliana

Evolutionary dynamics of the MAM locus. The organisation of the MAM cluster in Arabidopsis thaliana accessions is highly variable. Partial or complete deletions of MAM2 or MAM1 have occurred frequently. Also, partial gene conversion has occurred between loci. The predominant aliphatic glucosinolate class phenotype resulting from the structure of the MAM locus is indicated in the right column (3C: homomethionine-derived, 4C: dihomomethionine-derived) (Benderoth et al. 2009)
The logical next questions are: Which processes maintain the diversity? Why did the positively selected MAM1 gene not completely replace the ancestral MAM2 gene? Most likely, it was because under particular conditions it is advantageous for a plant to carry the MAM2 gene instead of the MAM1 gene. For example, MAM2 provides an advantage against certain generalist herbivores because MAM2 activity is associated with larger quantities of glucosinolates. Thus, balancing selection maintains both variants. The composition of local herbivore communities varies considerably over time. The relative frequency of generalists, specialists, parasitoids, etc., temporally changes within a given habitat. With these changes, different glucosinolate cocktails are beneficial and therefore are selected by the herbivore pressure.
8.4 Parasitic Plants

Parasitic plants. a Hemiparasitic mistletoes on host trees (Photo: copyright obtained from Shutterstock). b The hemiparasite Striga flowering in a maize field
Molecular insight regarding the interaction with host plants is largely restricted to parasitic plants sensu stricto and therefore only these will be discussed further. One reason for this bias is most likely the economic damage caused by parasitic plants such as Orobanche and Striga (Fig. 8.33), predominantly in Africa, where Striga infestation alone affects about 300 million farmers. Consequently, molecular understanding is mostly derived from studies involving species of these two genera.

Life cycle of Striga. (Yoshida and Shirasu 2012)
Other critical abilities of parasitic plants, besides haustoria-mediated penetration of host tissue, are the recognition of host plants and the establishment of nutrient transfer from the host. The first step is germination of seeds in response to signals that indicate the vicinity of a suitable host. The seeds of Striga and Orobanche are tiny and thus provide very little reserves for the developing seedlings. Early contact with a host is therefore essential. Striga and Orobanche locate hosts through highly sensitive detection of compounds exuded by the roots of such plants. Many structurally diverse molecules have, over the years, been shown to act as germination stimulants. Those best studied by far are the strigolactones (Fig. 8.35), a class of carotenoid-derived molecules found in small concentrations in root exudates (Fig. 8.35). They were isolated as germination stimulants for Striga seeds, hence the name strigolactones (Xie and Yoneyama 2010). For a long time it was not clear why a plant would exude compounds that compromise its performance by attracting enemies. This mystery was solved when strigolactones were first identified as indispensable signals for the interaction with mycorrhizal fungi (Chap. 7) and later as plant hormones that control shoot branching, root architecture and other developmental processes. Perception of strigolactones is thus an excellent example of how parasites or pathogens evolve the ability to use an essential molecule produced by potential hosts as a cue for the presence of such hosts.

Chemical structures of strigolactones and karrikins

Convergent evolution of strigolactone receptors. Homologues of the KAI2 gene are found in basal lineages such as charophytes. The strigolactone receptor D14 probably arose from these ancestral genes before the evolution of spermatophytes. Additional duplications of related karrikin receptor genes gave rise to rapidly evolving variants in parasitic plants that acquired the ability to detect strigolactones. Thus, strigolactone receptors evolved twice from more ancient karrikin receptors. The second event represents one of the steps towards parasitism. KL KAI2 ligand, D gene duplication. Question marks indicate uncertainty about receptor ligands. (Conn et al. 2015)
Like germination, the next step—haustoria formation—is triggered by chemical signals originating from the prospective host. Again, a range of molecules has been found to show the respective bioactivity, among them flavonoids, quinones and phenolic acids. Perception mechanisms, however, have not been molecularly elucidated yet. Invasion of host tissue is then reliant on localised destruction of host cellular structures by secreted cell wall–degrading enzymes and proteases. Finally, haustoria develop further and connect either with the xylem and phloem (holoparasites) or only with the xylem (some hemiparasites such as Striga). Symplastic connections with host cells enable the acquisition of organic carbon and nitrogen, as well as the exchange of macromolecules (including DNA and RNA) between the parasitic plant and the host plant.
The host ranges of most parasitic plants are rather broad. For example, the majority of Striga species parasitise a variety of grasses. Still, there is some degree of specialisation, as a few Striga species parasitise dicots instead. Also, many other plant species are not colonised, demonstrating the existence of non-host resistance against parasitic plants. The underlying mechanisms, however, are not clear. Penetration of host tissue should elicit wound and defence responses. Whether parasitic plants—in a manner similar to fungal and bacterial pathogens—suppress host defences by effectors that would in turn be recognised by hosts is not clear.
8.5 Allelopathy
When one plant species inhibits the growth of another species through the production of chemicals, this is commonly referred to as allelopathy. The original definition dating back to Hans Molisch in 1937 has since been broadened to include also beneficial interactions (positive allelopathy). In accordance with the “molecular stress physiology” perspective, we will discuss only negative allelopathy—that is, a plant–plant interaction that is harmful to one of them.

Intraspecific allelopathy in Kalanchoe daigremontiana. a Same-age daughter plantlets were placed in sand soil at regular intervals from the mother plant. After 150 days the height of the daughter plants clearly shows the inhibitory effect of the mother plant on their growth. b Quantification shows that the inhibition of the young plants depends on the distance from the mother plant. (After Bär et al. (2000))

The invasive plant Alliaria petiolata (garlic mustard) inhibits plant growth by disrupting symbiotic associations between native canopy tree seedlings and below-ground arbuscular mycorrhizal fungi. The extracts of garlic mustard (gm), sugar maple (sm), red maple (rm), white ash (wa) or a water control were tested for their effects on a mycorrhizal colonisation of native tree seedlings, b biomass gain of native tree seedlings and c percentage germination of native arbuscular mycorrhizal fungi spores. The bars represent the mean and standard error (Stinson et al. 2006)
8.6 Summary
-
Plants are constantly under attack by a wide range of potential enemies—namely, pathogens and herbivores. Still, most plants remain healthy because they possess an array of constitutive and inducible defence mechanisms.
-
Defences against pathogens and herbivores can both be divided into mechanical/physical and chemical defences. Also, both involve recognition events and signalling cascades that result in the induction of defence mechanisms upon the perception of a potential foe or the damage caused by it.
-
Plant pathogens are found among viruses, bacteria, fungi, oomycetes and nematodes.
-
Pathogens have to enter plant tissues, effectively suppress the plant’s immune system, gain access to the plants resources and be able to grow and reproduce rapidly within plant tissues.
-
Viruses are transmitted by phloem-sucking insects and spread through plasmodesmata. Bacteria have to enter a plant passively through stomata or wounds. Fungi and oomycetes can actively penetrate a plant’s surface.
-
Phytopathogenic bacteria, fungi and oomycetes employ effectors and toxins to interfere with the plant’s immune system and to disturb physiological or developmental processes.
-
The interaction between a plant and a pathogen can have two principal outcomes: incompatible when no disease arises, or compatible when the pathogen is able to propagate. In the former case the plant is called resistant, in the latter case it is called susceptible. A disease-causing pathogen is called virulent.
-
Constitutive defences are mechanical (e.g. cell walls, the cuticle) and chemical. Plants produce thousands of secondary metabolites with antimicrobial activity.
-
Inducible defences are mounted upon recognition of a potential pathogen.
-
Two principal amplitudes of defence based on two different recognition strategies can be differentiated. The first layer of inducible defence is referred to as innate immunity. Antimicrobial compounds (phytoalexins) are synthesised, cell walls are locally reinforced and various defence proteins are produced. These responses provide efficient protection against most potential pathogens.
-
Innate immunity is activated by the perception of molecules characteristic of potential pathogens (e.g. highly conserved bacterial structures) or characteristic of the activities of potential pathogens (e.g. cell wall fragments). Plant cells express >100 different pattern recognition receptors mediating this perception. Together they constitute an effective surveillance system that enables plant cells to sense the extracellular presence of many different latent foes.
-
Recognition triggers signal transduction cascades involving phosphorylation, Ca2+ signals and the production of reactive oxygen species in the oxidative burst.
-
The second layer of inducible defence is activated when virulence factors (effectors) are detected by plants. A cell undergoes a programmed cell death programme (the hypersensitive response), which limits the spread of the pathogen. The underlying signal transduction is poorly understood. Effector recognition requires large sets of resistance genes (R genes) because effectors are not conserved and pathogens produce a wide variety of effector molecules. R genes are plant species-specific and even genotype-specific. The evolutionary relationship between effectors (encoded by so-called avirulence genes) and R genes is described by the gene-for-gene hypothesis. Matching genes lead to an incompatible interaction (hence avirulence genes).
-
The ability of plants to keep pace with rapidly evolving pathogens in the evolutionary race is explained by the diversity of R genes in plant populations and the guard hypothesis. Many R gene products recognise not effector proteins but, rather, the modifications caused by effectors on sensitive virulence targets. Genetic diversity within a population reduces the vulnerability to pathogens.
-
Plants mount not only a localised response to pathogen attack but also a systemic response resulting in systemic acquired resistance. Long-distance signalling conveys the information about a pathogen attack to uninfected organs. The defence hormone salicylic acid is a key player in the systemic activation of defence.
-
The main immune system against viruses in plants is based on gene silencing mediated by small RNAs derived from double-stranded RNAs produced during virus replication. Immunisation against viruses is based on the systemic spread of gene silencing.
-
Insect herbivores are often referred to as pests. Three categories can be differentiated: chewing insects, piercing–sucking insects and phloem-feeding insects. A second fundamental distinction in addition to the feeding style is that between generalist herbivores (which do not show much preference for certain plant species) and specialist herbivores. The latter find their hosts through chemical cues and have evolved the ability to overcome the herbivore defences of their preferred hosts.
-
Constitutive herbivore defences include mechanical barriers, morphological features such as thorns and, most importantly, a diverse cocktail of chemicals that render a plant toxic or unpalatable. Major compound classes implicated in herbivore defence are alkaloids, phenolic compounds, terpenoids, cyanogenic glucosides and glucosinolates. Many defence molecules are stored in a non-toxic form as glycosides and are degraded to toxic aglycones upon tissue damage. In addition, plants produce non-proteinogenic amino acids, protease inhibitors and other defence proteins such as carbohydrate-binding proteins.
-
Inducible defences are both local at the site of herbivore-inflicted damage and systemic. Metabolism is reprogrammed and the synthesis of defence molecules is up-regulated.
-
An additional layer of herbivore defence is indirect defence. Many of the plant secondary metabolites or their respective breakdown products are volatile and influence a plethora of organisms in the ecological communities around a plant. For example, they can attract predators or parasitoids of the herbivores. Another form of indirect defence is based on the provision of rewards (extrafloral nectar) for predators.
-
Plant volatiles emitted by damaged plants can have yet another community consequence. They can result in the so-called priming of neighbouring plants. Perception of airborne signals indicating a herbivore attack on a plant leads to an up-regulation of defence in as yet uninfested plants or to more rapid and robust defence activation when these plants are attacked by herbivores.
-
Induction of direct and indirect herbivore defences is dependent on reliable cues perceived by the plant. A plant responds not only to wounding but also distinctively to signals associated with the insect’s activity. Sources of such signals can be insect saliva, regurgitant, oviposition fluids or faeces.
-
Jasmonic acid (JA) and its derivatives are plant hormones with a central role in balancing a plant’s investments in growth versus defence and specifically in protection from attack by insect herbivores and necrotrophic fungi. Loss of JA synthesis or JA perception severely compromises the ability of plants to limit damage caused by herbivores.
-
JA-dependent induction of herbivore defence proceeds in several steps: recognition of signals derived from herbivore attack; signal transduction involving transient cytosolic Ca2+ spikes, membrane potential changes, reactive oxygen species as signalling molecules and mitogen-activated protein kinase cascades; activation of JA biosynthesis; JA perception; and expression of herbivore defence genes.
-
JA synthesis proceeds from the ubiquitous fatty acid linolenic acid via enzymatic reactions localised in plastids and peroxisomes. The actual receptor-active JA–isoleucine (JA-Ile) conjugate is synthesised in the cytosol.
-
JA-Ile interacts with the receptor COI1. Upon binding, the E3 ligase complex (which COI1 is part of) ubiquitinates JAZ repressor proteins for proteasomal degradation. Early JA response genes can then be transcribed.
-
JA or JA conjugates are phloem-mobile signals that may travel to as yet unaffected tissues and activate the systemic wound response. In addition, volatile methyljasmonate serves as an airborne long-distance signal. An alternative mode of long-distance signalling could be electrical.
-
The interaction of plants with herbivores is a major driver of biodiversity and specifically of the diversity of secondary metabolism. Indeed, selection experiments have demonstrated evolution in action—that is, rapid changes in the composition of populations, depending on the strengths and nature of herbivore pressure.
-
About 1% of all dicot angiosperm species are parasitic. Two separate classes of parasitic plants have been defined: holoparasites are completely dependent on host photosynthesis, while hemiparasites are photosynthetically active at least during some stages of their life cycle and sometimes draw only water and mineral nutrients from the host. Parasitic plants can be further divided into root parasites and stem parasites.
-
An essential element of parasitism is access to the vascular system of the host. Parasitic plants penetrate host tissues with specialised structures called haustoria. These grow towards the vasculature and establish connections between the parasite and the host.
-
Plant parasitism has evolved several times independently. One key innovation was the evolution of highly sensitive receptors for germination stimulants exuded by plant roots—for example, strigolactones.
-
Allelopathy is the inhibition of one plant species by another plant species through the production and release of toxic chemicals. For several invasive plant species it has been proposed that part of their success is attributable to toxins in root exudates. However, few examples for clear molecular evidence of such interactions exist.