
Magnesium deficiency in spruce (Fichtelgebirge, Oberwarmensteinach, Germany): With insufficient Mg available in the soil, young needles obtain their Mg by re-translocation of Mg from the old needles via the phloem. Thus, the old needles become golden yellow at the time of bud break of new growth in spring. Excess of nitrogen enhances this process, especially when nitrogen is absorbed directly from the atmosphere. (Photo: E.-D. Schulze)
11.1 Availability of Soil Nutrients and Ion Use
11.1.1 Plant Nutrients
Typical concentration of nutrients in plant tissues (Marschner 1988)
Nutrient type |
Element |
Average concentration (μmol g−1) |
---|---|---|
Macronutrients |
N |
1000 |
K |
250 |
|
Ca |
125 |
|
Mg |
80 |
|
P |
60 |
|
S |
30 |
|
Micronutrients |
Cl |
3 |
B |
2 |
|
Fe |
2 |
|
Mn |
1 |
|
Zn |
0.3 |
|
Cu |
0.1 |
|
Ni |
0.001 |
|
Mo |
0.001 |
|
Beneficial elements |
Si |
11,000 |
Na |
200 |
|
Co |
0.1 |
Macro- and micronutrients are essential for the life of plants. Macroelements participate directly in metabolism (N, S and P) or indirectly support metabolism (K), and there are transitions to trace elements (Ca, Mg). Most micronutrients are metals, which are required for enzyme reactions (Chap. 7). Facultative or beneficial elements are required only in some plant groups (Si for grasses). Iodine and vanadium are not mentioned further here, as they are needed only in lower plants (Marschner 1988, 2012).
Concentrations of nutrient elements are often expressed in units of mass (grams per gram of dry weight). However, the physiological turnover and physico-chemical effects of these elements are usually measured not as mass but by the number of required molecules; therefore, the mole is a more relevant unit than the gram, but the gram remains as a measure of biomass. In contrast to the element concentration in biomass (moles or grams per gram of dry weight), in the field of plant ecology the element content quantifies the amount of nutrients per area (moles or grams per square metre of leaf or of ground area), which takes into account the bulk density and the profile depth in soils or the thickness of leaves (grams per square metre).
This chapter deals with the essential macronutrients. Molecular aspects of plant responses to nutrient scarcity and excess, transition metals as trace elements and stress caused by toxic elements are considered in Chap. 7.
11.1.2 Availability of Nutrients in Soil
Rocks are mixtures of different minerals and are classified according to their chemical composition and how they were formed. A distinction is made between crystalline rocks (formed from the cooling of molten magma in the Earth’s crust (intrusive rock) or on the Earth’s surface (igneous rock)), sedimentary rocks (originating from deposition of weathered material or of chemical degradation products by sedimentation and subsequent hardening) and metamorphic rocks (formed by partial re-melting and recrystallisation of crystalline and sedimentary rocks).
11.1.2.1 Primary Minerals

Mineral composition of crystalline rocks and formation of clay minerals. a Composition of important rocks with respect to their base and Si content. b Conversion of primary minerals into secondary clay minerals. (Blume et al. 2010)
Tetrahedron structures of primary silicates
Primary silicate |
Free charges per tetrahedron |
Tetrahedra structure |
Minerals |
---|---|---|---|
Nesosilicates SiO4 4− |
4 |
Tetrahedra are cation saturated |
Olivine (Mg, Fe)2[SiO4] |
Sorosilicates Si2O7 6− |
3 |
Few tetrahedra covalently bound |
Diopside (Ca, Mg)[Si2O6] |
Inosilicates SiO3 2− |
2 |
Tetrahedra form a chain |
Augite (Ca, Mg, Fe, Al, Na)[Si2O6]n |
Cyclosilicates [Si2O6]1.5− |
1.5 |
Tetrahedral chains form ribbons |
Amphibolite (Na, K, Ca, Mg, Fe, Al)(OH4)[Al2–4Si14–12O44] Tourmaline NaMg3Al6[(OH)1(BO3)3 [Si6O18] |
Phyllosilicates Si2O5 2− |
1 |
Tetrahedra form layers |
Muscovite (KAl2)(OH2)[Si3AlO10] Biotite K(Mg, Fe, Mn)3(OH, F)2[AlSi3O10] |
Tectosilicates SiO2 |
No free charges 1 free charge with Al replacing SiO2 |
Quartz SiO2 Orthoclase feldspar K[AlSi3O8] Anorthite Ca[Al2Si2O8] |
The free charges of various primary silicates, as listed in Table 11.2, are the basis for weathering and cation availability for roots. It is mainly the substitution of Si with Al that determines the actual cation content of the rock and the proportion of elements (Fe) that may be oxidised. A series of increasing stability with weathering (olivine → augite → tourmaline → feldspar → quartz) emerges. Basalt, for example, is much more alkaline than granite—that is, it contains more potassium, calcium and magnesium—and thus it weathers faster.
11.1.2.2 Secondary Minerals
-
Two-layered minerals: 1 Si tetrahedral + 1 Al octahedral layer: Kaolinite, serpentine, with no cations in the intermediate layers.
-
Three-layered minerals: 1 Si tetrahedral + 1 Al octahedral + 1 Si tetrahedral layer: Illite, chlorite, vermiculite, montmorillonite. Cations occur in the intermediate layers, and these cations can be reversibly exchanged against hydrogen ions.
The clay minerals allophane and imogolite are exceptions. They occur exclusively in soils derived from volcanic activity. They have a spherical or tubular structure, and they can adsorb anions in acid soils (e.g. phosphate) in such a tight manner that they are no longer available to plants.
Oxides and hydroxides: In parallel to the breakdown of the Si tetrahedrons during silicate weathering, oxidation of metals contributes to the breakdown of the primary crystal structure. The most important oxides and hydroxides are those of Fe (particularly those of Fe2+ to Fe3+ or Fe hydroxide) and of Mn and Al. Depending on climatic conditions, these oxides form different types of secondary minerals, which are often responsible for the characteristic colouring of soils. Tropical soils are often bright red as a consequence of haematite (α-Fe2O3), while the yellow-brown colour of many soils of the temperate zone is caused by goethite (α-FeOOH).
-
Rocks: sandstone (70% Si), greywacke (67% Si), slate (59% Si), limestone (8% Si).
-
Loose sediments: drifting sands (97% Si), loess (72% Si), marl (64% Si).
Depending on the original rock and its “weathering history”, sedimentary deposits have different cation contents. Clay-rich soils possess a greater capacity for exchange of cations than sandy soils not only because of their much larger surface area for ion exchange but also because of the greater number of covalent bonds. Secondary minerals are included in sedimentary rocks; for example, limestone contains also aluminium oxide, iron oxide and manganese (hydr)oxide.

Global distribution of important soil types (Compiled by G. Guggenberger)
The cation exchange capacity (CEC) of a soil is a measure of the amount of cations that can be reversibly stored. It is large in clay minerals that swell (e.g. montmorillonite: 80–120 cmolc kg−1 (c = charge)) and small in non-swelling clay minerals (e.g. kaolinite: 1–10 cmolc kg−1) (see Box 11.1) (Osman 2013). The chemical composition of the original rock or of the sediments determines the availability of water and nutrients (soil fertility) and thus the type of vegetation. Plant cover may increase the CEC of soils by a factor of 10 by formation of “humus” as a product of incomplete decomposition of organic matter. But even at high humus contents in soil, the chemical composition of the original rock determines the type of humus formed and thus the availability of nutrients in the soil: humus has a potential CEC between 100 and 300 cmolc kg−1, where “c” expresses the fact that the number of charges is counted depending on the soil pH (Osman 2013). Thus, the availability of cations in the soil affects most ecosystem processes.
11.1.3 General Aspects of Plant Nutrition
11.1.3.1 Nutrient Supply in the Soil Solution
In mineral soils, chemically characterised by the content of alkaline cations (base saturation: e.g. K, Mg and Ca) and physically determined by the texture (particle size distribution), roots act as additional ion exchangers. While cations in the soil are in equilibrium between the exchanger (clay minerals, oxides, humus) and the free soil solution, roots cause chemical imbalances because of active transport processes, which enable plants to take up cations against the concentration gradient. This process is in most cases selective for certain ions. In exchange against cations from the soil, the root actively releases H+, HCO3 − or organic acids to the soil, which originated from the carbon cycle (Jones et al. 2004). The uptake of alkaline cations in exchange for protons results in acidification of soils. With uptake of anions, alkalinisation of soils may occur. If the vegetation is not managed or harvested, these minerals are returned in the dead biomass to the soil as litter. In agriculture and forestry, nutrients are removed from the soil by harvesting, grazing or use of litter, with the consequence that the chemical conditions in the soil change (acidification of soil water, podzolisation) if the depletion exceeds the resupply by weathering. Deposition into the soil of strong acids from the atmosphere, as a result of air pollution (SO4 2−, NO3 −), has an effect similar to that of harvesting of plant material, because the input of these anions is not balanced by cations. Fertilisation and liming of soils balance the loss of cations caused by harvesting or leaching.
Box 11.1: Ion Exchange Capacity of Soils
Soils: Soils are the product of weathering and transportation of original materials of the Earth’s continental land surface by physical and chemical processes and biological agents. They are mixed to different extents with dead organic matter, litter and humus.

Dependence of the effective cation exchange capacity (CECeff) on soil pH. The cation exchange capacity is shown for average conditions of a soil with 20–30% clay content and 2–3% humus content. The potential cation exchange capacity (CECpot) includes, in addition to the basic cations, the dissociable protons. (Blume et al. 2010)
-
Arable fields: marshland (37 cmolc kg−1), pelosol (22 cmolc kg−1), black earth (18 cmolc kg−1), para brown earth (17 cmolc kg−1)
-
Forest: brown earth (60 cmolc kg−1), pseudogley (15 cmolc kg−1)
Even though, energetically, uptake of anions and cations is fundamentally different, both ions are taken up via transporters and ion channels (Chap. 7). There are specific interactions between cations and anions; for example, in the presence of Cl−, uptake of K+ decreases (see Marschner 2012).
11.1.3.2 Ion Uptake by Roots
Roots grow in soils as part of the rhizosphere (see Box 11.2), where decomposition of organic material interacts with ion and water uptake by roots. The root surface is covered by microbial and fungal communities. Water uptake and ion uptake (see Box 11.3) take place primarily through younger parts of the root and near the meristems of emerging adventitious roots. In addition, ions may be taken up or lost via leaves and shoots. Above-ground uptake of ions plays an important role during uptake of air pollutants (Burkhardt et al. 2012; Harrison et al. 2000). Along the root, uptake of nutrients changes with root development, as transport depends on the ion radius and chemical composition. Root uptake occurs either in the cell wall (the apoplast) or in the cytosol (the symplast) (Chap. 7). During radial transport from the soil to the central stele, the apoplast is composed of cell walls of cells outside the endodermis. At the endodermis, all ions must pass through the symplast of specialised transmission cells. Besides this radial transport pathway, uptake of ions may also take place through the meristems, where the endodermis is not yet formed. The partitioning of ion uptake between the symplast and apoplast remains under debate. For water it has been estimated that 10–70% of uptake occurs via the apoplasmatic pathway (White 2012); this flow of water may carry a major fraction of Ca, Na and Al through the apoplast into the xylem, and these ions may accumulate in leaves (White 2012). Nevertheless, for metabolic use, these ions must also enter the symplast. In contrast, uptake and transport of P, K, N and Mg occurs in the symplast. Ion transport decreases when the secondary root cortex is developed.
Box 11.2: The Rhizosphere (Contribution by Else K. Bünemann)
The rhizosphere is defined as the volume of soil around living plant roots that is affected by root activities such as root growth, water and nutrient uptake, and exudation of organic anions. The efflux of carbon from roots via lysates from damaged cells and exudates from intact cells (rhizodeposition) is typically 10–20% of net photosynthetically fixed carbon. This carbon stimulates beneficial or pathogenic microorganisms around the roots; this was first observed by Lorenz Hiltner (1862–1923), who coined the term “rhizosphere effect”. The key players in the rhizosphere include the plant; saprophytic organisms such as bacteria, archaea, fungi and invertebrates; and symbiotic microorganisms such as rhizobia and arbuscular mycorrhizal fungi. The interaction of these key players with each other and with the soil environment is called rhizosphere ecology.
Nutrient uptake by plant roots occurs mainly in the root hair zone and leads to the depletion of nutrients close to the root. The extent of the depletion zone depends on the mobility of the nutrient in the soil. For example, the depletion zone of nitrate may extend 40 mm away from the root, while the depletion zone of phosphate typically extends only 2 mm. Phosphorus uptake in barley has been shown to be positively related to root hair length, indicating the importance of root hairs in nutrient uptake. In addition, arbuscular mycorrhizal fungi can transport phosphorus over a 10–15 cm distance. Root hairs and arbuscular mycorrhizal fungi can also increase the drought resistance of plants.
Changes in pH in the rhizosphere result from the secretion of protons or hydroxide ions by plants when they are taking up an excess of cations or anions, respectively, in order to keep their cytosolic pH constant and balance net charges. Mobilisation of iron by secretion of phytosiderophores can be beneficial not only to the secreting plant but also to a neighbouring plant that cannot produce phytosiderophores but can take up the iron–phytosiderophore complex. Secretion of carboxylates such as citrate and malate in order to solubilise phosphorus is sometimes accompanied by secretion of phenolics and cell wall degrading enzymes, which reduce the microbial degradation of the secreted carboxylate.

Roots of a winter wheat plant pulled out of the ground in spring. The soil that sticks to the root is called the rhizosheath. This binding of soil to the root is largely due to root hairs. Note the root tips that are not yet covered with soil, because of the absence of root hairs in this root zone. In the field, rhizosphere soil can be obtained for further analysis by uprooting a plant, shaking off the non-adhering soil and collecting the adhering soil either by brushing it off or by immersion in a suitable liquid. (Photo: E.K. Bünemann)
-
Growth (all nutrients).
-
Catalysis (e.g. Fe in oxygenases, Zn in hydrogenases).
-
Transport of electrons (Cu, Fe, Mn).
-
Accumulation (Chap. 12 for definitions) of ions where supply exceeds demand.
-
Storage and reserve (e.g. N, P).
Concentration of substances in the xylem and phloem of Nicotiana glauca (Schurr and Schulze 1995). The concentrations do not indicate the amount that is transported, because the mass flow is the result of the concentration and the flow rate. The flow in the phloem is slower than that in the xylem. Organic acids were not measured
pH and substances |
Phloem (μg ml−1) |
Xylem (μg ml−1) |
---|---|---|
pH |
7.8–8.0 |
5.6–5.9 |
Sucrose |
170–196 |
1.1–1.2 |
Amino substances |
10,808 |
283 |
Potassium |
3673 |
204 |
Phosphorus |
435 |
68 |
Chlorine |
486 |
64 |
Sulphur |
139 |
43 |
Magnesium |
104 |
34 |
Sodium |
116 |
46 |
Calcium |
83 |
189 |
Ammonium |
45 |
10 |
Zinc |
16 |
2 |
Iron |
9 |
0.6 |
Copper |
1 |
0.1 |
Manganese |
0.9 |
0.2 |
Nitrate |
<1 |
70 |

Schematic model of the cycling of potassium and the uptake of nitrate in the xylem and phloem. In the phloem, K is the counter-ion for transport of malate and sugars. In the root, K is released from the phloem and transported into the xylem, where it is the counter-ion for nitrate. Nitrate uptake occurs both by co-transportation with potassium from the soil solution and by an exchange with HCO3 −. (Marschner 1988)
Box 11.3: Ion Uptake (see White 2012)
-
Diffusion: Ion gradients lead to passive transport in soils and in the cell wall (Al3+)
-
Uniport: An H+ gradient leads to passive transport via ion channels (K+)
-
Symport: H+ may be transported back with anions (Cl−, SO4 2−)
-
Antiport: H+ efflux causes a cation influx (K+, Ca2+, NH4 +)
In a plant cell, ion exchange occurs not only on the outer membrane surface but also at the membranes of plastids and vacuoles. Large differences in cation demand occur between organelles. Chloroplasts have high Mg concentrations, while mitochondria have high Ca concentrations. The vacuole serves as an ion reservoir. The pH of the cytosol is stabilised by the continuous exchange of cations and/or anions (a pH stabilisation mechanism, pH-stat). In this process, K+ is important for balancing the exchange of H+. Thus, K+ and H+ regulate the cellular proton concentration and proton gradients (Chap. 7)
-
is oxygen-dependent
-
can be inhibited by mercury if the ion channels or carriers are lined with S-containing amino acids
-
is temperature dependent in the case of carriers, with an optimum generally at 30 °C
-
is suited to the external concentration; that is, there are ion uptake systems that are most efficient at high or low external ion concentrations. Transporters with low efficiency for substrate ions can also have a function as sensors (Marschner 2012)
-
is subject to competition in the presence of other ions; for example, the uptake of Mg2+ decreases in the presence of K+ or Ca2+
-
Elements transformed in metabolism: They change the level of reduction (e.g. N and S).
-
Elements that cannot be metabolised but support metabolism: These are freely moving cations (K+, Ca2+, Mg2+) and anions (PO4 −) that store energy, or metals with catalytic functions that are bound firmly to proteins and act chemically, for example, because of changes in their oxidation state (Fe, Mn, Cu and others).
Analysis of the nutrient balance of the plant shows that a large part of nutrients is continuously exchanged within the plant; that is, ions circulate between the root and the shoot. This applies particularly to individual organs that have a limited life-span. Root tips and leaves are periodically or continuously formed newly, utilised transiently and disposed afterwards. Obviously, leaves are time-limited “machines”. Old leaves are shed in response to decreasing physiological activity, for example, when shaded by new leaves. At the same time, formation and shedding of leaves are mechanisms for acclimation of the whole plant to changing environmental conditions (particularly light). Leaf shedding often also serves other functions, for example, detoxification following excessive uptake of salt (e.g. Na).
The expanding leaf is first a sink for carbohydrates and nutrients. Organic substances are required for the structure of the leaf. In the young state, leaves are thus a carbohydrate sink (Chap. 12); that is, sink leaves import carbohydrates for their growth. The leaf becomes a source leaf only after the development of about a third of the leaf area, exporting carbohydrates for maintenance or growth at other sites. Even before full development of the leaf area, a leaf’s capacity for photosynthesis decreases and finally it becomes unproductive. This process of leaf ageing is modified by environmental conditions, including nutrition and light. In contrast to ageing, senescence is a genetically regulated process of the fully differentiated cell (Guarente and Kenyon 2000; Thomas et al. 2000). Ageing and senescence finally lead to shedding of leaves. However, before shedding, part of the invested resource of nutrients is remobilised and transported back into the plant. Large variations exist between species and between types of nutrients. Thus, the leaf is a “flow-through system” for nutrients, highly adjustable to environmental conditions and the internal requirements of the plant for nutrients. The turnover rate of nutrients in plants may change suddenly, depending on the ontogeny of the whole plant, particularly during the change from vegetative to reproductive growth.
11.1.4 Nutrient Deficiency and Excess

Typical nutrient deficiencies and damage due to excess or deficits of nutrients. a Nitrogen deficiency. b Manganese deficiency. c Iron deficiency. d Potassium deficiency. e Magnesium deficiency. f SO2 damage. g Road salt damage. h Mild phosphate deficiency. i Severe phosphate deficiency. (Hartmann et al. 1988)
-
Nitrogen deficiency: Evenly distributed chlorosis on the whole tree and for all needle ages; small needles, compressed sprouting, growth inhibition. Usually not limited to individual trees. On moor and heathland soils. Possibilities of misidentification: honey fungus (Armillaria spp.), but here individual trees or younger needles turn yellow.
-
Manganese deficiency: Light yellow chlorosis of younger needles, starting in the lower canopy, particularly on limestone. Mn is not phloem mobile; that is, requirements for growth are met only by root uptake and influx in xylem water. Therefore, with increasing age, symptoms disappear.
-
Iron deficiency: Whitish-yellow chlorosis of the youngest needles. Considerable deficiency causing needle tips to brown and die on limestone. Similar to Mn deficiency.
-
Potassium deficiency: Pale yellow to violet-brown (reddish) colouration starting from needle tips, but at first predominantly on older needles, which are shed prematurely and lead to light canopies. K is phloem mobile and is thus first retransported to the youngest organs. K deficiency increases sensitivity to frost. Misidentification: Mg deficiency, but in this case the colouration is more intensely yellow or, rarely, red.
-
Magnesium deficiency: Light yellow to golden-yellow chlorosis, starting in older needles from the tip. Shaded branches and the underside of needles are less decolourised. Mg is phloem mobile and therefore is remobilised from old to young needles. Occurs on silicate soils.
-
Air pollution (SO 2) damage: Golden-brown to reddish-brown colouration, starting from the needle tip. Necrotic flecks or bands in the middle part of the needle. Buds often die off. Misidentification: damage from road salt, herbicides.
-
Road salt damage: Needles of the previous year’s growth are dark copper brown in spring, falling in early summer. Buds often fall. Damage along the edge of roads. Misidentification: frost-drought (but here, the needles are light reddish brown) or sulphur dioxide.
-
Phosphorus deficiency: Starting with a dark blue-green colour (increased formation of chlorophyll), later violet-red to copper-brown and yellow colouration (anthocyanin), in parts spotty, starting from the edge of the leaf. Misidentification: K deficiency, drought, salt, but then without the violet-red colour.
11.2 Nitrogen Nutrition
11.2.1 Nitrogen in Plant Metabolism
-
Amino acids (free amino acids, proteins).
-
Nucleic acid (DNA).
-
Heterocyclic and azo-compounds (e.g. a pyrrole ring).
The huge amount of N2 in the Earth’s atmosphere is very inert and not directly available to plants. Nitrogen can be used only in its reduced or oxidised form (NH4 + or NO3 −) or in its organic form (-NH2). In nature, the transformation of gaseous N2 into nitrogen available for plants occurs either in the atmosphere through lightning (10–40 mol N m−2 year−1), by free-living bacteria in the soil, or in plant-associated nitrogen-fixing bacteria. Industrially reduced nitrogen for fertilisers is synthesised by the Haber–Bosch process from N2 and H2 at high temperatures and high pressures (Chap. 21).
The process of N 2 fixation occurs catalytically under anaerobic conditions in root nodules of legumes or in free-living cyanobacteria (blue-green algae; see Marschner 2012), where the microorganisms lower the oxygen concentration of the atmosphere via respiration. In the primary succession from open soil to vegetation with a closed nitrogen cycle, there is a stage at which N2-fixing organisms dominate (Chap. 17). Because of the high energy demand for N2 reduction (and the requirement for P associated with it), these organisms are unable to compete at the later stages of succession, when more nitrogen is available in oxidised and reduced forms (Read 1993). Here we concentrate on N uptake and turnover in plants.
11.2.2 Nitrogen Uptake and Nutrition
Plants gain nitrogen from litter and soil solution as amino acids, ammonium cations and nitrate anions. From the atmosphere, N is available as NH3, NO or NO2 gas. Leguminosae may gain nitrogen also by symbiosis with soil bacteria.

Schematic model of the coupling of the photosystem and the cell metabolism of nitrogen. a Coupling of electron transport from photosystem I (PS I) to sulphite, nitrite and nicotinamide adenine dinucleotide phosphate (NADP) reduction via ferredoxin. b Diagram of the coupling of C and N metabolism in a plant. For a detailed description, see the text. (Marschner 1995)
11.2.2.1 Amino Acid Uptake and Nutrition
Amino acids are available from N2 fixation by nitrogen-fixing bacteria, via the direct degradation of litter or from the soil solution (Wallenda et al. 2000). It is known from laboratory and field experiments that roots are able to take up a broad spectrum of amino acids (Näsholm et al. 2009).
Amino acid uptake by roots is very important, particularly in boreal forests at a low rate of nitrification (Persson et al. 2000). Under these conditions, amino acid uptake via the mycorrhiza supplies the plant with almost all of its N demand. Mycorrhizal fungi are able to excrete proteases and thereby break down proteins from litter and use the resulting amino acids directly (Näsholm et al. 1998; Wallenda et al. 2000). It has been shown (Nordin et al. 2001; Persson et al. 2006) that the uptake of amino acids also takes place in the presence of ammonium and nitrate ions, and may reach the same level in fertile soils and at poor sites (Berthrong and Finzi 2006), even though the relative contribution to the N requirement is lower at fertile sites, as additional ammonium and nitrate are available and are utilised (Schulze et al. 1994; Michelsen et al. 1996).
11.2.2.2 Ammonium Uptake and Nutrition
Ammonium is taken up directly in the area of the root hairs or via mycorrhizae. NH4 + would be toxic as an ion because its ionic radius and strength are very similar to those of K+. Thus, it could enter the cell instead of K+, interfering with the pH regulation of the cell. Therefore, NH4 + taken up by the root is not stored or transported but rapidly converted into amino acids via the GOGAT (glutamine oxoglutarate aminotransferase) enzyme system. In this process, NH4 + is bound to glutamate, using adenosine triphosphate (ATP). The primary amino acid glutamine is formed, which donates the NH2 group to oxoglutarate by transamination and thereby regenerates glutamate. GOGAT is the enzyme system that also traps free NH4 + ions in all other plant organs.

Schematic model of the exchange and transport of ammonium. Protons are released to neutralise ionic charges when NH4 + is taken up; that is, the soil pH decreases (Fig. 11.9b). Ammonium is immediately assimilated into amino acids in the roots; for this reaction, C skeletons have to be transported from the shoot to the root. Ammonium-fed seedlings are deficient in soluble carbohydrates, as the C skeletons are used for assimilation of NH4 + in the roots and are thus not available for growth of shoots. Detoxification of ammonium leads to a surplus of amino acids, which are not used for protein synthesis, as the assimilates in the roots are required for the assimilation of ammonium ions. This effect is not apparent with nitrate, because nitrate can be stored in the vacuole in balance with growth. (Modified from Marschner (1988))
11.2.2.3 Nitrate Uptake and Nutrition

Schematic model of the exchange and transport of nitrate. a Nitrate uptake and transport in the plant. To balance the charges, OH− ions are released into the soil; that is, the soil pH rises. Nitrate can be stored in the vacuole until reduction (Marschner 1988). b Changes in soil pH after nitrate or ammonium uptake. Roots of plants were divided between two compartments: in one, the N source was nitrate and in the other it was ammonium. The yellow colouration shows the decrease in pH in proximity to the root during ammonium uptake. The red colour shows nitrate uptake and the corresponding pH increase. (Photo courtesy of E. George)
Physiologically, nitrate is not toxic and thus it may be stored in the root or in the shoot. Nitrate storage in the leaf has a regulatory (signal) effect on C allocation (shoot–root growth) of plants (Scheible et al. 1997a, b; Klein et al. 2000). A high nitrate concentration in the leaf stimulates shoot growth and inhibits root growth by regulating the sugar transport to the root, whereas in the soil it stimulates root growth.
Generally, nitrate transport occurs via the xylem into the storage parenchyma of the stem, or into the leaf where nitrate is initially stored together with cations in the vacuole. This increases the osmotic concentration and the water content of leaves (e.g. in “crunchy” nitrate-fertilised vegetables—mainly salad vegetables). If required, nitrate may be transported back from the vacuole into the cytosol. This occurs in exchange with organic acids formed via PEP carboxylase in the leaf. For some plant species, oxalic acid is transported, in exchange for nitrate, into the vacuole. At high Ca supply, this leads to the formation of Ca oxalate in the vacuole. Because of the low solubility of Ca oxalate, crystals are formed in the vacuole, which may even damage the cell structure (rhaphides, giving the typical taste of rhubarb and banana peel). Nitrate is reduced to nitrite in the cytosol. Since nitrite is toxic, it must be reduced to NH4 + rapidly, which is then assimilated by the GOGAT system. The rate of turnover of nitrite reductase is faster than that of nitrate reductase. Some of the cations taken up with nitrate are transported back into the phloem; in particular, K+ is relocated into the root, accompanied by associated organic acids. These acids are decarboxylated in the root (e.g. malate) and thus support nitrate uptake.
11.2.2.4 Ammonium Nitrate Nutrition
-
Maintains the balance of anions and cations in the plant and in the soil.
-
Decouples the uptake of cations and anions from the N nutrition (the N requirement is larger than the requirement for cations).
-
Maintains the C/N balance. The relation of nitrate and free amino acids (in the case of ammonium nutrition) to starch is a sensitive indicator of the state of nutrition in the leaf (Fig. 11.10). Large nitrate and amino acid concentrations are always connected and indicate excess fertilisation. In contrast, high starch concentrations in the morning correlate with small nitrate and amino acid concentrations and thus indicate N deficiency. Maximum growth rates are achieved under conditions where the nitrate and starch concentrations safeguard the supply for growth during the night, because growth and thus the N and C requirement continue during the night, although assimilation of N and C requires light.

Relations between nitrate, starch in leaves and plant growth. a Relative growth rate (RGR, shown as isohypses) in relation to nitrate and starch concentrations in the leaf. The highest growth rates occur at lower concentrations of the two substances. Starch accumulation is thus an indicator of N deficiency and nitrate accumulation is an indicator of C deficiency. b Consumption of nitrate reserves of plants grown in different nutrient conditions, following a sudden decrease in N availability. Even in plants that are well supplied with nitrate, the supply of nitrate from reserves lasts only a few days because of the high growth rate of Raphanus. (Stitt and Schulze 1994)
11.2.2.5 Nitrogen Input from the Atmosphere
Nitrogen-containing air pollutants are taken up as gas (NH3, NO, NO2) by the plant via the stomata. NH3 is more soluble than NO, which in turn is more soluble than NO2. In the leaf, all gases are immediately assimilated into amino acids. Not only gases but also ions dissolved in rainwater and fog reach the inside of the bark via the medullary rays (Klemm 1989). Dust particles containing Mn are deposited on the outside of the cuticle, catalyse the oxidation of NO2 and SO2 to nitrate and sulphate in a surface reaction and thus increase N deposition. Uptake of nitrogen from the atmosphere contributes significantly to the N balance for growth in the canopy of trees (up to 20–40% of the N requirements) (Harrison et al. 2000; Burkhardt et al. 2012).
There is a basic difference between the N uptake via the shoot and that via the root. Uptake of nitrogen via the root is metabolically regulated; that is, the plant takes up nitrogen according to its requirements. In contrast, the plant has no “defence” mechanisms against uptake of NOx, NO2 or NH4 + via the shoot. In addition, uptake via the shoot occurs in exchange with cations (K, Mg); that is, it is coupled with a loss of cations. Nitrogen nutrition from air pollutants thus may lead to an imbalance in cations, particularly if cation uptake is limited.

Soybean field in Brazil (Photo courtesy of M. Hertel)
11.2.3 Nitrogen Requirements for Growth
Nitrogen nutrition is important not only for plants growing under natural conditions but also for crops. The requirements and responses of woody plants are different in magnitude to those of herbaceous plants, because wood contains very little nitrogen. Not only the growth form but also the season determine the requirements for N.

Changes in the grain yield of maise with different N and P fertilisation. At high P availability (140 kg P ha−1), 300 kg N ha−1 can lead to a high yield. If only 40 kg P ha−1 is available, then addition of only 160 kg N ha−1 leads to loss of yield. (Schopfer and Brennicke 1999)
Herbaceous species are better equipped to utilise nitrate than woody species. Herbaceous species usually have smaller dry weights and greater water content per leaf area (Schulze et al. 1994), and they are better able to store nitrate because of their larger vacuoles. Because of greater N concentration in the mesophyll (and thus a greater concentration of the CO2-reducing enzyme RubisCO), herbaceous plants usually have higher rates of photosynthesis and thus an greater capacity for nitrate reduction than woody plants (Fig. 11.7). A further basic difference between herbaceous and woody species is the association of herbaceous plant roots with vesicular arbuscular mycorrhizae (VAMs) and ectomycorrhizae (EMs) in woody plants. VAMs are more able to utilise mineralised P, while EMs are able to gain amino acids from litter (Smith and Read 1997). EM bypass mineralisation (Wallenda et al. 2000; Kähkölä et al. 2012). Ectomycorrhizae are also able to take up ammonium in acid soils, as they precipitate the toxic Al with phosphate. There are also mycorrhizae that reduce nitrate (Wallenda et al. 2000). Detection of whether plants utilise nitrate, ammonium or amino acids is partly possible via stable isotopes (Hobbie et al. 2000; Persson et al. 2006). The N requirement for growth (C/N relation) of fungi is higher than the N requirement of trees, as the cell wall of fungi consists of chitin (50 mg N g−1 dry matter for fungi and 1 mg N g−1 dry matter for wood). However, there are exceptions: Fraxinus spp. use large amounts of nitrate but do not possess any mycorrhizae. In trees, the nitrate is reduced in the mycorrhizae or in the roots, so nitrate can no longer be detected in the xylem water.
In ecology, a theory has been developed that species with a high material turnover are stress sensitive and those with a low turnover are stress tolerant concerning water supply (Orians and Solbrig 1977; Fig. 12.5). This could, however, not be supported for N supply and its interaction with growth by Fichtner and Schulze (1992). There was no difference in the response to reduced nitrogen between species that are adapted to high N supply (e.g. Galeopsis tetrahit (common hemp nettle (Labiatae) at eutrophicated sites)) and species adapted to poor N supply (e.g. Teesdalia nudicaulis (shepherd’s cress (Cruciferae) on sandy lawns)). The important difference between the two species was that Teesdalia flowered at low N supply, when Galeopsis was able to set flowers only at high N supply (Chap. 12).
11.2.4 Nitrogen Storage
-
As inorganic nitrogen in the vacuole, only in the form of nitrate and together with cations. This storage balances the changing requirements during the diurnal rhythm of growth and during the changing seasonal nitrogen demand and supply.
-
As organically bound nitrogen stored in the form of amino acids and proteins. Most of these substances have further functions; that is, storage is a by-product of metabolism and of an imbalance in production and consumption of certain materials. Amino acids serve as storage and transport forms for N even though amino acids act osmotically. Therefore, amino acids with several –NH2 or –NH groups are stored (glutamine (2 N/4 C), asparagine (2 N/4 C), arginine (4 N/6 C), allantoin (4 N/4 C). Storage is short-term to seasonal and occurs in the phloem–xylem circulation or in the vacuoles of the storage parenchyma of stems. Some of the amino acids may not only have a storage function but also protect against frost and salt in winter (proline; Chaps. 6 and 7). The storage amino acids play an important ecological role in sprouting and growth of young leaves in spring. Thitithakanul et al. (2012) found that up to 45% of the total N stored during the previous year is mobilised before bud break in young poplars. Polypeptides and proteins are formed when the osmotic activity of amino acids may interfere with metabolism; for example, during the loading of phloem the number of molecules must be decreased and N must be concentrated in a few molecules (Schulze et al. 1999). Important storage proteins (e.g. prolamine, glutelin and albumin) accumulate in seeds and may also have protective functions. The CO2-reducing enzyme RubisCO (ribulose-1,5-bis-phosphate-carboxylase/oxygenase) forms about 30% of the N content of a leaf. Only a proportion of this protein is normally active—with the excess serving to safeguard the photosynthetic apparatus at high photon flux—or a proportion may be degraded during N deficiency and used for growth (Stitt and Schulze 1994). Before leaves are shed, part of this nitrogen is degraded and the amino acids are re-translocated back to the plant. This remobilisation of nitrogen from leaves may be as high as 20% of the leaf N (Chapin and Kedrovski 1983; Koch et al. 1988).
11.2.5 Insectivorous Plants
-
Spring traps (e.g. Dionaea).
-
Pitfall traps (e.g. Nepenthes).
-
Sticky traps (e.g. Drosera).
-
Suction traps (e.g. Utricularia).

Distribution of insectivorous plants in angiosperms. Different forms of leaf are used to capture insects. Spring traps: Dionaea muscipula; pitfall traps: Darlingtonia californica and Nepenthes spp.; sticky traps: Drosera spp.; suction traps: Utricularia spp. (Modified from Schulze (1991))
Contribution of insect N to the N nutrition of insectivorous plants (Schulze et al. 2001)
Trap type |
Genus |
Growth form |
Proportion of insect N in plant N (%) |
---|---|---|---|
Sticky trap |
Drosera |
Rosette |
20 (e.g. D. erythrorhiza, Western Australia) |
Climber |
53 (e.g. D. macrantha, Western Australia) |
||
Upright, low |
48 (e.g. D. stolonifera, Western Australia) |
||
Upright, high |
54 (e.g. D. gigantea, Western Australia) |
||
Roridula |
Shrub |
70 |
|
Pitfall trap |
Cephalotus |
Rosette |
26 |
Nepenthes |
Climber |
62 (100% in buds) |
|
Darlingtonia |
Rhizome |
76 |
|
Heliamphora |
Rhizome |
79 |
|
Brocchinia |
Erect rosette |
59 |
|
Spring trap |
Dionaea |
Rosette |
80 |
Roridula gorgonias (from South Africa) is a special case, as it does not excrete proteases. The sticky leaves catch insects, which are then harvested by bugs (Hemiptera) that are adapted to life on the sticky trap and move slowly and carefully. Roridula uses the excrement of the bugs as a N source (Ellis and Midgley 1996).
Nepenthes takes nitrogen from the trapped insects in the form of ammonium, which is absorbed via glands (Schulze et al. 1997), where amino acids are formed before transport to the vascular bundle. There, polypeptides are formed (a N concentration mechanism) and then loaded into the phloem. In young Nepenthes shoots, 100% of the N content originates from trapped insects.
In the Venus flytrap (Dionaea), survival depends on the seedlings being able to trap insects (Schulze et al. 2001). Only after a significant catch can the larger spring traps develop that are suited to catching even larger insects; only the rosette is capable of flowering.
11.2.6 Nitrogen Deficiency and Excess
-
N deficiency: Yellowing, reduction of growth of shoots and leaves, high dry weight/leaf area, starch accumulation, premature ageing and shedding of leaves. Yellowing of leaves is not very specific and is often also caused by deficiency of other nutrients and degradation of chlorophyll. The nitrogen–cation imbalance determines deficiency and excess.
-
N excess: High chlorophyll content, high water content, low dry weight/area (shade leaf type), nitrate and amino acid storage, long internodes.
Because of the rapid and substantial alterations in N supply with changing conditions in time and location, it is very time and resource consuming to quantify the N supply. In contrast to nitrate, which is not bound to a soil exchanger and can thus be measured in the soil solution, quantifying the exchangeable ammonium in litter is difficult. In order to characterise the supply of nitrogen at a particular site, Ellenberg (1974) suggested deriving the supply from the presence of certain indicator species at a certain site. Each species was classified by a relative scale, with a range of 1–5 to 1–9 as indicator values (Ellenberg 2009) (see Box 11.4). Conversely, it is possible to draw conclusions from the occurrence of a certain species about the supply of resources at a certain site. The scaling of the indicator value is valid only in a limited region, as the interaction with other species changes the occurrence and thus the indicator value of a particular species (Chap. 19).
Box 11.4: Indicator Plants
-
About 2000 vascular plants in central Europe occur with varying frequency in different habitats and are classified into particular classes and locations. This classification is also applied to mosses and lichens
-
The classification is based on three climatic factors (light, temperature and continentality) and four soil factors (N availability, soil pH, soil water content and salinity). Development of a classification of species in relation to P supply is in progress
-
These values are the so-called indicator values and should allow general characterisation of a site, without additional analysis in a laboratory
-
The values increase from 1 (small) to 9 (large)
-
Indicator values are not a measured value but based on expert knowledge. They are extrapolated from the normal behaviour of a species in its natural environment; that is, they do not characterise the physiological requirements but the ecological niches they occupy. Thus, they apply only in areas where the initial floristic analysis was performed
-
N1 species only occur in N-poor environments (e.g. Trifolium arvense, Erophila verna, Calluna vulgaris)
-
N2 and N3 species occur more often in N-poor locations than in N-rich locations (e.g. Medicago sativa, Equisetum arvense)
-
N4 and N5 species occur in moderate to rich locations but only infrequently in N-poor locations (e.g. Ribes nigrum, Primula vulgaris)
-
N6 and N7 species flourish only in N-rich locations (e.g. Chenopodium album)
-
N8 species are special N indicators (e.g. Mercurialis annua, Urtica urens, Epilobium angustifolium, Ballota nigra)
-
N9 species occur in extremely N-rich locations (e.g. Chenopodium bonus-henricus, Lamium album, Sambucus nigra)
11.3 Sulphur Nutrition
11.3.1 Sulphur in Plant Metabolism
-
in its oxidised form as SO 4 2− ion (sulpholipids are ester bindings of SO4 to a sugar lipid; these occur in all membranes and as an -S–S or -S–O group, which occurs in species-specific secondary metabolites—for example, alliin in Allium and isothiocyanate R-N-C-S in Brassicaceae). These substances serve as S storage and protection against herbivory.
-
in its reduced form as—SH groups in amino acids (cysteine, methionine), as a co-enzyme (acetyl-S-enzyme protein: acetyl-CoA), as a non-peptide unit making a functional protein (prosthetic group of ferredoxin, thiamine, biotin), in proteins to form the tertiary structure by oxidation of the disulphide, in cellular redox systems (R1–SH + R2–SH → R1–S–S–R2 + H2, particularly with participation of glutathione), in proteins to bind heavy metals (heavy metal resistance; Chap. 7, where cysteine participating in ion channels may bind to heavy metals (Hg)) and in the glutathione cycle to detoxify radicals.
11.3.1.1 Sulphur Metabolism

Schematic model of the assimilation of sulphur into various plant metabolites. (1) sulphate esterase, (2) sulphate reductase, APM adenosine phosphomalate, APS adenosine phosphosulphate, ATP adenosine triphosphate, PAPS phosphoadenine phosphosulphate. (Marschner 1988)
11.3.2 Sulphur Uptake and Plant Requirements
Sulphur uptake as the SO4 2− anion is a symport with protons. Sulphate is the most important additional anion, after nitrate and phosphate, in plant nutrition. Reduction of the sulphate anion to —SH occurs in the chloroplast, via sulphite utilizing the electron flow from PS I (Fig. 11.7). With calcium, sulphate forms crystals (gypsum). Thus, sulphate cannot be stored in its inorganic form in the vacuole.
Generally, the requirement for N also regulates the demand for S, as the N to S ratio in proteins is approximately constant (10:1). Only in some plant families does the S requirement increase because of secondary metabolism. This applies particularly to the Cruciferae, where mustard oil (glucosinolate) provides protection against herbivory but also serves as an S reserve. Rape seed belongs to those species that grow well at very high S supply. Since mustard oil is volatile, these plants are also able to remove S via stomata by emission of mustard oil (it can be smelled when rape seed is flowering). The S requirement is also large in Leguminosae, particularly because of the S-containing storage proteins in seeds.
Sulphur is taken up not only via the roots but also via the shoot. This particularly applies to sulphur dioxide (SO 2), which enters the intercellular spaces together with CO2. SO2 is soluble in the cell wall with formation of the toxic sulphite anion, which follows the pH gradient from the cytosol to the chloroplast, where it is assimilated. In general, SO2 is oxidised to SO4 2− by heavy metals in the cuticle and in the cell wall, particularly by Mn. Thus, airborne sulphate is assimilated in the mesophyll in the same way as sulphate that was taken up by the roots. Sulphate may react in the cell wall with Ca and form gypsum crystals. Because of its toxicity, the toxic sulphite formed from SO2 after dissolution in cell wall water must be reduced and sequestered in amino acids rapidly.
Transport of S in the xylem of trees and from the atmosphere in healthy and damaged spruce trees (Köstner et al. 1998)
Healthy stand (mmol m−2 soil year−1)a |
Damaged stand (mmol m−2 soil year−1)a |
|
---|---|---|
Sulphate |
25.9 |
34.7 |
Glutathione |
0.52 |
0.47 |
Cysteine |
0.14 |
0.14 |
Methionine |
0.3 |
0.3 |
Uptake of SO2 |
7.4 |
7.6 |
Total S load for the canopy |
34.26 |
43.21 |
11.3.3 Indicators of Sulphur Deficiency and Excess
-
S deficiency: The symptoms are similar to those of N deficiency (chlorosis), with accumulation of non-S-containing amino acids (arginine, aspartate) and inhibition of protein synthesis.
-
S excess: High SO2 stress leads to the well-known pollution (smog) damage. Lichens are particularly sensitive. The damage is caused by the formation of sulphite and accumulation in the chloroplast. The threshold for SO2 damage is 5 μg m−3 as an average concentration in the air.
11.4 Phosphate Nutrition
11.4.1 Phosphorus in Plant Metabolism
-
Structural functions: P participates in the formation of DNA and RNA as a bridge between the ribose-N bases, and in the formation of phospholipids of membranes.
-
Metabolic functions: Energy is stored and transferred by polyphosphate esters with adenosine. Di- and tri-esters are involved in the actual energy storage in the cell (adenosine monophosphate (AMP), adenosine diphosphate (ADP), adenosine triphosphate (ATP)) and energizing of binding sites for metabolic turnover. Sugar phosphates (e.g. fructose-1,6-diphosphate) play a decisive role as substrates and, as substrates in the regulation of sugar metabolism and triosephosphates, are an important transport metabolite between the chloroplast and cytosol (Stitt 1994).
11.4.2 Phosphate Uptake and Plant Requirements
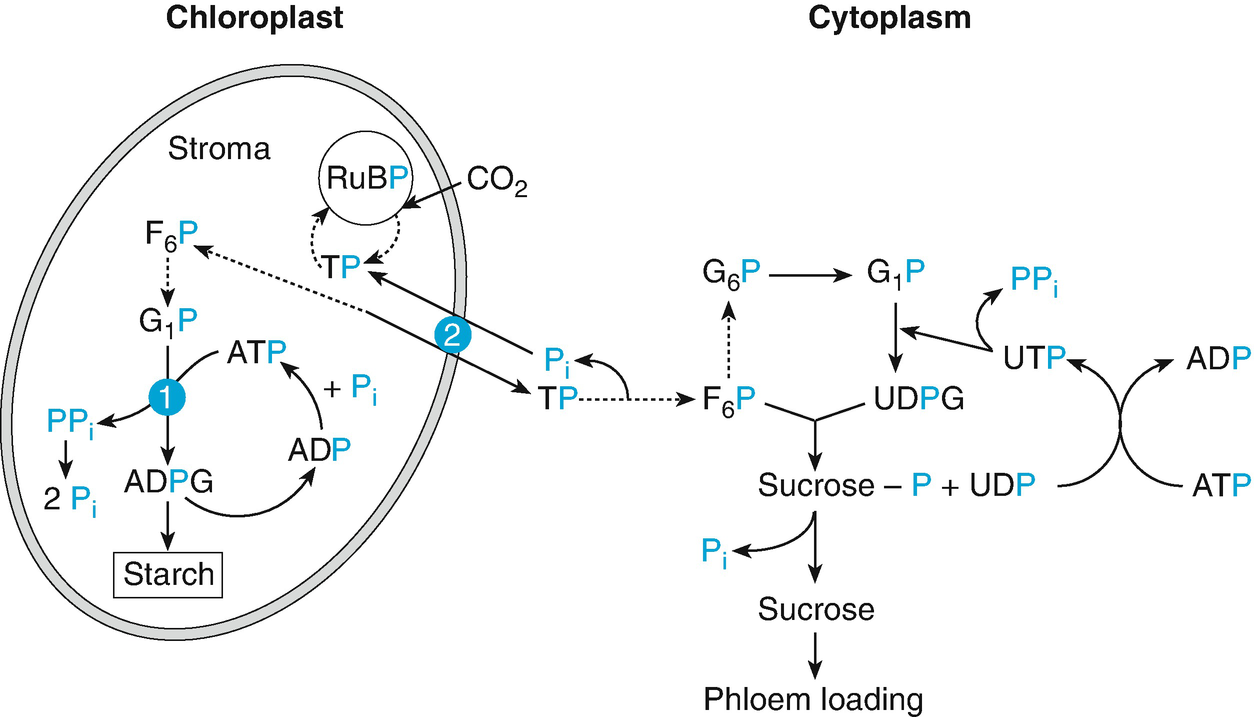
Schematic model of the role of phosphate in the synthesis of starch and sucrose, and in regulating the metabolism of plants: adenosine diphosphate (ADP)–glucose pyrophosphorylase regulates starch synthesis: inhibition by inorganic orthophosphate (Pi) and stimulation by 3-phosphoglyceric acid (PGA) (1). Phosphate translocation regulates the export of photosynthetically produced substrates from the chloroplast: stimulation by Pi and triosephosphate (TP) (2). F 6P fructose-6-phosphate, G 6P glucose-6-phosphate. (Marschner 1988)
Phosphate is stored in plants in the form of polyphosphates or as sugar esters; in phytate, up to six phosphate molecules may be deposited on one sugar (myoinositol). This form of storage is very important in seeds.
11.4.3 Indicators of Phosphorus Deficiency and Excess
-
P deficiency: This causes interference in reproductive processes (delay in flowering), reduced longitudinal growth, dwarfing, smaller leaves because of reduced cell growth, increased root growth, dark green colouration of leaves and reddish colouring of needles, with chlorosis and premature ageing (Fig. 11.6).
-
P excess: This does not occur naturally, because phosphate is not easily dissolved and may be regulated by polyphosphate formation.
11.5 Alkaline Cation Nutrition
The alkaline elements potassium, magnesium and calcium are essential macronutrients, which are not metabolised but are essential to provide the correct environment for particular reactions, to regulate pH and to regulate cellular water relations.
11.5.1 Magnesium
11.5.1.1 Function of Magnesium
-
Structural functions: In the structure of chlorophyll (the central atom of four pyrrole rings) and in the ether connections of cellulose fibrils in cell wall pectins.
-
Metabolic functions: In stabilizing enzymes, predominantly during the turnover of phosphates (nitrogenase, ATPase, phosphorylase and others). In these processes, Mg2+ binds to phosphate and, because of allosteric interactions, binding between the substrate and phosphate becomes possible. Mg2+ is involved in stabilizing energy-rich molecules—for example, phytate as phosphate storage. Mg2+ is a counter-ion for proton production during photosynthesis in the chloroplast (pH 7.6 in the dark and 8 with illumination). Mg2+ participates in the osmoregulation and pH regulation in the cell.
11.5.1.2 Uptake and Requirement for Magnesium
In the soil, magnesium is bound to the substrate predominantly as an exchangeable cation. Mg2+ is a component of primary and secondary minerals (serpentine), from which it is released by weathering. The most important antagonist for Mg2+ is Ca2+, but also NH4 +, K+, Mn2+ and even H+ influence the uptake of Mg2+. In the plant, magnesium is transported in the xylem and phloem, and the ion is stored in chloroplasts. Remobilisation from ageing leaves occurs.
11.5.1.3 Magnesium Deficiency and Excess
-
Mg deficiency: Yellowing of older leaves, yellow tips and early shedding of needles, chlorosis due to inadequate chlorophyll synthesis, starch accumulation because of the effect on phosphate metabolism, altered water relations due to poor osmoregulation, dwarf growth (Fig. 11.6).
-
Mg excess: On Mg-rich substrates (serpentine) because of interactions with other nutrients, water stress may lead to increased concentrations of Mg.
Box 11.5: Interactions of Magnesium, Nitrogen and Aluminium During Forest Decline

Magnesium and N concentrations in healthy and damaged spruce. a Seasonal change (shown on the x axis) in the magnesium concentration of spruce twigs that, prior to treatment, had Mg deficiency symptoms, in comparison with healthy green twigs. In the damaged and undamaged twigs, either buds were removed and thus growth was halted, or buds were maintained unchanged. Damaged side shoots without buds showed an increase in the Mg concentration during the year and were green at the end of the growing season. This experiment was decisive in showing that yellowing of spruce grown in silicate soils was a direct consequence not of SO2 but of the interaction between growth and Mg supply. Mg deficiency is also clearly visible on the spruce canopy on the title page of this chapter (Lange et al. 1989b). b Changes in magnesium concentration with N concentration in twigs of spruce before emergence (top); translocation of Mg from old to new needles (centre) and after emergence of young needles (bottom). The change is shown in twigs with high and low Mg supply. Only spruce grown in conditions of high N availability and low Mg supply cross the threshold that leads to yellowing. (Oren and Schulze 1989)
11.5.2 Calcium
-
Structural function: Stabilisation of the cell wall, together with Mg.
-
Metabolic functions: Activator of membrane-bound enzymes (amylase) and ATPases, and osmoregulation (Ca oxalate). Ca2+ interacts with phytohormones (indole-3-acetic acid (IAA) and auxin) during elongation of cells (calmodulin) and with abscisic acid (ABA). Ca2+ is transported in the cytosol by linking to proteins. Ca2+ functions in the mitochondria, in contrast to Mg2+, which regulates the proton gradient predominantly in chloroplasts.
11.5.2.1 Uptake and Requirement for Calcium
Ca occurs in the soil solution as Ca2+. Calcium-rich soils are predominantly found on limestone (Box 11.6). The pH of these soils with almost unlimited CaCO3 is stabilised by the dissolution of CaCO3 at about pH 7. In Ca-poor soils, CaCO3 is quickly consumed because it dissolves easily and is not stable against weathering, so the dissolved Ca2+ is bound to the ion exchangers in the soil only after the dissolution of the carbonate. The pH of Ca-poor soils is usually around 5 and may decrease on acidic rocks (shales containing pyrite) to 3.5. Below a pH of 5.5 the chemistry of the soil is increasingly determined by aluminium. Ca2+ uptake at the root is antagonistically influenced by other cations, particularly Al at low pH. If Al instead of Ca2+ is incorporated into the cell wall, elongation growth is inhibited.
Ca2+ uptake balances other anions, particularly nitrate and sulphate. It occurs predominantly at the root tip, where the endodermis is not yet formed. Transport into the xylem via the cell wall is possible. Ca2+ is transported in the xylem, but it is absent from the phloem. Because of the high pH in the phloem, Ca2+ would react with phosphate, forming insoluble apatite. But Ca2+ is transported in the phloem bound to a protein (e.g. calmodulin). Ca2+ is stored in cell walls and vacuoles together with malate, where Ca oxalate, Ca sulphate or Ca carbonate may be formed by precipitation. High Ca concentrations also occur in the endoplasmic reticulum.
Box 11.6: Indicator Plants for Soil pH Conditions
-
R1 + R2: Strong acidity indicators; they never occur on slightly acidic to alkaline soils (e.g. Scleranthus annuus, Rumex acetosella, Spergula vernalis)
-
R3 + R4: Acidity indicators, mainly on acidic soils, but they also occupy soils of neutral pH (e.g. Raphanus raphanistrum, Stachys arvense, Anthemis arvense)
-
R5 + R6: Moderate acidity indicators; they seldom occur on strongly acidic soils or on neutral to alkaline soils (e.g. Sinapis arvensis, Veronica persica, Fumaria officinalis)
-
R7 + R8: Weak acidic to weak basic indicators; they never occur on strongly acid soils (e.g. Aconitum napellus, Arctium lappa, Corydalis cava)
-
R9: Base and lime indicators; they only occur on alkaline soils (e.g. Delphinium consolida, Adonis aestivalis)
-
For limestone- and silicate-indicating plants (Part 4, Chap. 18)
Ca reaches the leaf in the xylem stream but may be transported out via the phloem only if bound to proteins, resulting in an excess and accumulation of Ca in the leaf over time. This becomes obvious from the Ca content of leaves, which rises with age and is particularly striking in conifers with needles of different ages (Oren et al. 1988).
The function of Ca as a stabiliser of the primary wall, by binding to pectin, becomes important in elongation growth, which essentially depends on the presence of Ca. However, Ca has further functions in growth, ranging from the formation of callose to the secretion of the calyptra and the regulation of geotropism. By forming bridges between phosphate and carboxyl groups of phospholipids, Ca maintains the membrane structure.
11.5.2.2 Calcium Deficiency and Excess
-
Ca deficiency: Disturbed growth by inhibition of cell division, desiccation of tips and buds, death of root tips and chlorosis of needle tips. Ca deficiency is possible during ripening of fruits and in organs that grow fast but transpire little (bulbs, tubers, taproots, etc.), as Ca is not transported in the phloem. In fruits, Ca deficiency leads to increased susceptibility to fungal attack.
-
Ca excess: Occurrence of Ca oxalate, Ca sulphate and Ca carbonate. Grasses generally require less Ca than dicotyledons. Low Ca requirements and adverse reactions to high Ca supply also occur in “calcifuge” plants, which are not able to maintain compartmentation of large amounts of Ca (Chap. 3).
11.5.3 Potassium
Potassium is the most required nutrient in plants (2–5% dry weight), after nitrogen, with the following metabolic functions (no direct involvement in chemical reactions): pH regulation, enzyme activation by changing the conformation of proteins (ATPase), influencing protein synthesis (translation, synthesis of ribulose-1,5-bisphosphate (RuBP) carboxylase), osmoregulation and elongation growth of cells, tropism and movement (stomata regulation), the main cation in phloem sap (Table 11.3).
These metabolic functions of potassium cannot be replaced by Na+ (the most important cation in animals). However, the osmotic function of K+ in the vacuole may be replaced by Na+, Mg2+ and Ca2+. The K concentration is high in the cytosol and low in the apoplast (except in movements and tropism: osmotically regulated movement—for example, of stomata). Transport through the membrane is via K channels.
-
Regulation of photosynthesis (photophosphorylation) and drought resistance: K+ determines the osmotic potential during drought and maintains growth with decreasing water potential (Fisher and Turner 1978). The effect of K+ on osmoregulation also explains its importance in frost resistance
-
Regulation of movements: Function of stomata (Chaps. 6 and 9). A corresponding mechanism affects other movements—for example, of the leaves of Mimosa pudica and Dionaea muscipula on touching, and the orientation of legumes to the sun
-
Phloem transport: Potassium is the most important cation regulating the pH in the phloem and sucrose loading
-
Regulation of fruit and tuber (potato) ripening
11.5.3.1 Uptake and Requirement for Potassium
Potassium occurs predominantly in silicate rocks and becomes reversibly bound to exchangers in the soil, particularly to clay minerals. Because of its ionic radius, potassium fits optimally into the intermediate layers of clay minerals and is therefore preferentially accumulated there (specific adsorption). In some soils, accumulation of K+ in clay minerals that are able to swell results in contraction of these clays, which thus “fix” K+ so strongly that it is no longer available for plants. The ionic radius and physical characteristics of K+ are similar to those of ammonium, so these two ions are easily interchangeable. An excess of ammonium leads to a loss of K+ (and other cations) and if these cations are leached, this stimulates acidification of soils. K+ and ammonium are both used to determine the cation concentration of the exchanger.
11.5.3.2 Potassium Deficiency and Excess
-
K deficiency: Reduced growth and increased remobilisation from ageing organs, disturbed water relations (drying of tips), wilting particularly at the edge of older leaves, yellowing and early shedding of needles (Fig. 11.6).
-
K excess: With K excess (e.g. on granite containing muscovite), Ca and Mg uptake are competitively influenced, thus leading to increasing Mg deficiency at these sites.
11.5.3.3 Potassium Accumulation by Mistletoes
Mistletoes are heterotrophic phloem parasites (leaves without chlorophyll), as well as autotrophic xylem parasites (with green leaves). Xylem parasites have been known for their medicinal use since Hippocrates’s time, and not just since Asterix and Getafix. Their alkaloids and lectins (carbohydrate-binding proteins) have major pharmaceutical relevance in the treatment of tumours (Lev et al. 2011). Mistletoes are of botanical interest (Calder and Bernhardt 1983) as they have a high potassium concentration in their leaves (40 mg g−1). In hyperparasitic plants (mistletoe growing on mistletoe), additional accumulation of K and other cations may occur (157 mg g−1 Na in arid locations) (Ehleringer and Schulze 1985) because the mistletoe takes up the xylem water with the cations that accumulate in the shoot, as there is no connection and recirculation to the host’s phloem. This accumulation of salt can lead to leaf damage and abscission. In mistletoes it is particularly significant that despite the high, almost toxic, ion concentrations, they maintain higher transpiration rates than their hosts (Ehleringer et al. 1985). Thus, mistletoes are not only significant sinks for cations but also water parasites (Glatzel and Geils 2009).
Characteristic nutrition of mistletoes and their hosts
Mistletoe |
Host |
Source |
|
---|---|---|---|
K concentration (mg g−1) |
28 |
11 |
(1) |
P concentration (mg g−1) |
2 |
1 |
(1) |
N concentration (mg g−1) |
28 |
25 |
(1) |
10 |
11 |
(2) |
|
Transpiration (mmol m−2 s−1) |
4 |
2 |
(2) |
CO2 assimilation (μmol m−2 s−1) |
4 |
5 |
(2) |

Leaf mimicry of Australian mistletoe (see Calder and Bernhardt (1983)): Acacia cambagei with Amyema maidenii. (Photo: E.-D. Schulze)
11.6 Summary
-
Plant nutrients are divided into (1) the macronutrients C, O, H, N, S, P, K, Mg and Ca; (2) the micronutrients Fe, Mn, Zn, Cu, B, Mo, Cl and Ni; and (3) the “beneficial” elements Na, Si, Co, I and V. The elements C, O, H, N and S are major constituents of organic matter. P esters are essential in energy transfer reactions. K, Na, Ca, Mg, Mn and Cl are involved in osmotic reactions, in enzyme reactions, in bridging reactions, and as balancing ions controlling membrane permeability. Fe, Cu, Zn and Mo occur as chelates in prosthetic groups of enzymes and enable electron transport by valency change.
-
The availability of nutrients is variable and dependent on the type of the original rocks, which determines the texture and the chemical composition of soils; climate, which controls weathering; vegetation, which selectively removes or returns certain elements; and management of the nutrient supply.
-
Nutrient uptake by plants occurs mainly via the roots but may also occur via shoots (leaves and bark), which is important in the presence of air pollutants.
-
Depending on the availability of nutrients or biotic pests, specific deficiency diseases may occur, which are recognisable by specific symptoms in the leaves.
-
Nitrogen is second to carbon in importance for plant life. N uptake occurs from the soil or from the air as ammonium, as nitrate, as amino acids, or as gaseous NH3 or NOx. A number of plant species can, together with symbiotic microorganisms, form nodules in which microorganisms assimilate N2 from the air into ammonia. Ammonia and ammonium ions are toxic to cells and are rapidly assimilated into amino acids. Ammonium uptake leads to acidification of the soil, C imbalance and cation deficiency. The uptake of nitrate is coupled with cation uptake, which leads to increasing pH (alkalisation) in the soil. Excess nitrate leads to soil acidification due to leaching of nitrate and associated cations. Indicator plants allow an assessment of the average N availability in ecosystems. Nitrogen deficiency leads to selection of plants that capture mainly insects and use them as N sources. Nitrogen deficiency can be beneficial at low cation availability.
-
Sulphur is required in plants in the oxidised form (sulphate ester), as well as in the reduced form (—SH groups of amino acids). Sulphate reduction occurs in chloroplasts. Uptake occurs predominantly in the form of sulphate via the roots. Crucifers have particularly high S requirements.
-
Phosphate serves as the carrier of chemical energy in the plant. Phosphate is not reduced in plants but is used in polyphosphate esters.
-
Basic cations are not metabolised but are important in enzymatic reactions and in reaction centres of enzymes (Mg in chlorophyll). The requirement for K is higher than that for Ca and Mg. K regulates osmotic concentrations and the pH of the cell. Mg is involved in chlorophyll functions and in the transfer of phosphate from adenosine triphosphate to organic molecules. Ca acts as an osmotic dehydrating ion and as a signal in cell metabolism (calmodulin).