
Water freezes not only on the surface of a plant at sub-zero temperatures but also in the plant tissues. Freezing-tolerant plant organs limit intra-organ ice formation to the intercellular spaces, extracting water from the cells, mainly their vacuoles. Aspects that are not well understood are the ice nucleation process and the significance of cellular components—in particular, special cell wall–bound proteins as potential catalysts of heterogeneous nucleation. Extra-organ ice may propagate—for example, via the stomata, hydathodes or lenticels—into the interior, triggering the freezing of extracellular water. (Photo: www.fotocommunity.de/photo/hagebutten-im-raureif)
4.1 The Temperature Challenge
Temperature is the second dominant factor (after precipitation) that determines the global structure of vegetation. On more than 60% of the continental surface of the Earth, frost can occur at least temporarily. Because of the fundamental importance of water for life, frost (i.e. temperatures below the freezing point of water) is a major threat for any type of organism. This applies in particular to poikilothermic organisms such as plants, which equilibrate with the temperature of their environment. Therefore, frost tolerance is a major factor for the distribution of the terrestrial vegetation on Earth. On the other side, even in the hottest regions (with the exception of volcanoes)—Al’Aziziyah in Libya and Death Valley in California, USA, with the highest ever recorded temperatures of 57.8 °C and 56.7 °C, respectively—higher plant life is possible, provided that moisture is available. Extreme temperatures are commonly accompanied by other stresses—in particular, a shortage of water, which is either frozen at sub-zero temperatures or simply not available in hot places.
4.1.1 Temperature Dependence of Life

Life processes of an organism, described as a function of temperature. The relative growth rate (R) or percentage of survival, respectively, may be used as a measure of life:
4.1.2 Plants as Poikilothermic Organisms
Only very few examples of plant organs that can produce heat by alternative respiration are known. One of these is the spadix of Aracean flowers. Plants are poikilothermic organisms, which cannot maintain their temperature at a level different from that of their immediate environment (Chap. 9). However, as the exterior temperatures can vary substantially for different organs such as roots (soil temperature) or leaves (air temperature, local heating by sunrays), temperatures within one individual plant can be very different at any given time. Furthermore, environmental temperatures can change rapidly. Thus, plant cells, tissues and organs require the ability to autonomously respond to temperature fluctuations.
4.1.2.1 Temperature Dependence of Metabolism

An increase in the temperature speeds up the reaction; a decrease slows it down. The Q10 is a direct indicator of the temperature dependence of a reaction. The Q10 for enzymatic reactions lies between 1.4 and 2.5, while for biophysical processes it is between 1.03 and 1.3. Both the range of Q10 values for enzymatic reactions and the difference between biochemical and biophysical reactions pose fundamental problems for plants.




Because of variations in the efficiency of catalysis, not all biochemical reactions that take place at the same time in a plant cell require the same activation energy. Therefore, a change in the temperature could easily disturb the metabolic balance in reaction chains—in particular, those involving steps with a high Q10. Plants must be able to compensate for potential temperature-caused metabolic imbalances—that is, changes of pool sizes of metabolites.

Temperature dependence of an enzyme-catalysed reaction. While at temperatures below the optimum the Q 10 rule is applicable, temperatures higher than the optimum progressively inactivate the enzyme by affecting its molecular structure (denaturation). Therefore, the temperature response curve of a biochemical reaction is asymmetrical with a strong decline towards higher temperatures
Since the cellular metabolism is composed of a large number of individual reactions whose reaction rates are dependent not only on the temperature but also on the equilibrium constants and sizes of the substrate pools, heat inactivation of the cellular metabolism is not as sudden as that of individual enzymes. Comparably strong inactivation does not take place at temperatures below the optimum.
4.1.2.2 Temperature and Photosynthesis
Practically any type of stress that impairs CO2 assimilation in the Calvin cycle under high light can cause oxidative damage and photoinhibition (of photosynthesis). Low temperatures are among the most prominent of these stresses (Chap. 12). Essentially, two processes account for oxidative damage. First, excitation energy cannot be channelled efficiently into the reduction of nicotinamide adenine dinucleotide phosphate (NADP+), because the balance is disturbed between photosynthetic thylakoid reactions (the so-called light reactions, which are virtually not decelerated), and the biochemical CO2 fixation reactions which, in contrast, are slowed down considerably in the cold. As a consequence, reduced NADP (NADPH) is not oxidised at a sufficient rate in the reducing phase of the Calvin cycle, leading to a shortage of the electron acceptor NADP+. Under such conditions, electrons are transferred to O2 instead and thereby radicals and reactive oxygen species (ROS) are produced. Depending on the scavenging capacity of the cell, ROS can be detoxified or cause damage. In addition, they can act as signals activating pathways that target genes for ROS-detoxifying proteins such as ascorbate peroxidase, glutathione reductase or superoxide dismutase (Chap. 2, Sect. 2.2).

Photoinhibition by chilling at a photon flux density (PFD) of about 450 μmol quanta m−2 s−1 upon exposure for 2.5 h to the indicated temperatures. The rate of photosynthesis by leaf slices maintained at 25 °C at the same PFD is 100%. a Photosynthetic CO2 net uptake. b Photosynthetic quantum yield of photosystem II (H2O → 2,6-dimethyl-p-quinone) and photosystem I (diaminodurene → methylviologen/O2), respectively, measured in potato leaves. Cucumber, common bean, tomato and potato are chilling sensitive; spinach is chilling tolerant (Sonoike 1996)
4.1.2.3 Membrane Fluidity
Chemical structures and melting points of the most common fatty acids in biomembranes
Carbon skeleton |
Structure |
Common name |
Melting point (°C) |
---|---|---|---|
16:0 |
CH3(CH2)14COOH |
Palmitic acid |
63.1 |
18:0 |
CH3(CH2)16COOH |
Stearic acid |
69.6 |
20:0 |
CH3(CH2)18COOH |
Arachidic acid |
76.5 |
16:1 (Δ9) |
CH3(CH2)5CH〓CH(CH2)7COOH |
Palmitoleic acid |
−0.5 |
18:1 (Δ9) |
CH3(CH2)7CH〓CH(CH2)7COOH |
Oleic acid |
13.4 |
18:2 (Δ9,12) |
CH3(CH2)4CH〓CHCH2CH〓CH(CH2)7COOH |
α-Linoleic acid |
−5.0 |
18:3 (Δ9,12,15) |
CH3CH2CH〓CHCH2CH〓CHCH2CH〓CH(CH2)7COOH |
α-Linolenic acid |
−11 |
20:4 (Δ5,8,11,14) |
CH3(CH2)4CH〓CHCH2CH〓CHCH2CH〓CHCH2CH〓CH(CH2)3COOH |
Arachidonic acid |
−49.5 |
4.1.2.4 Freezing
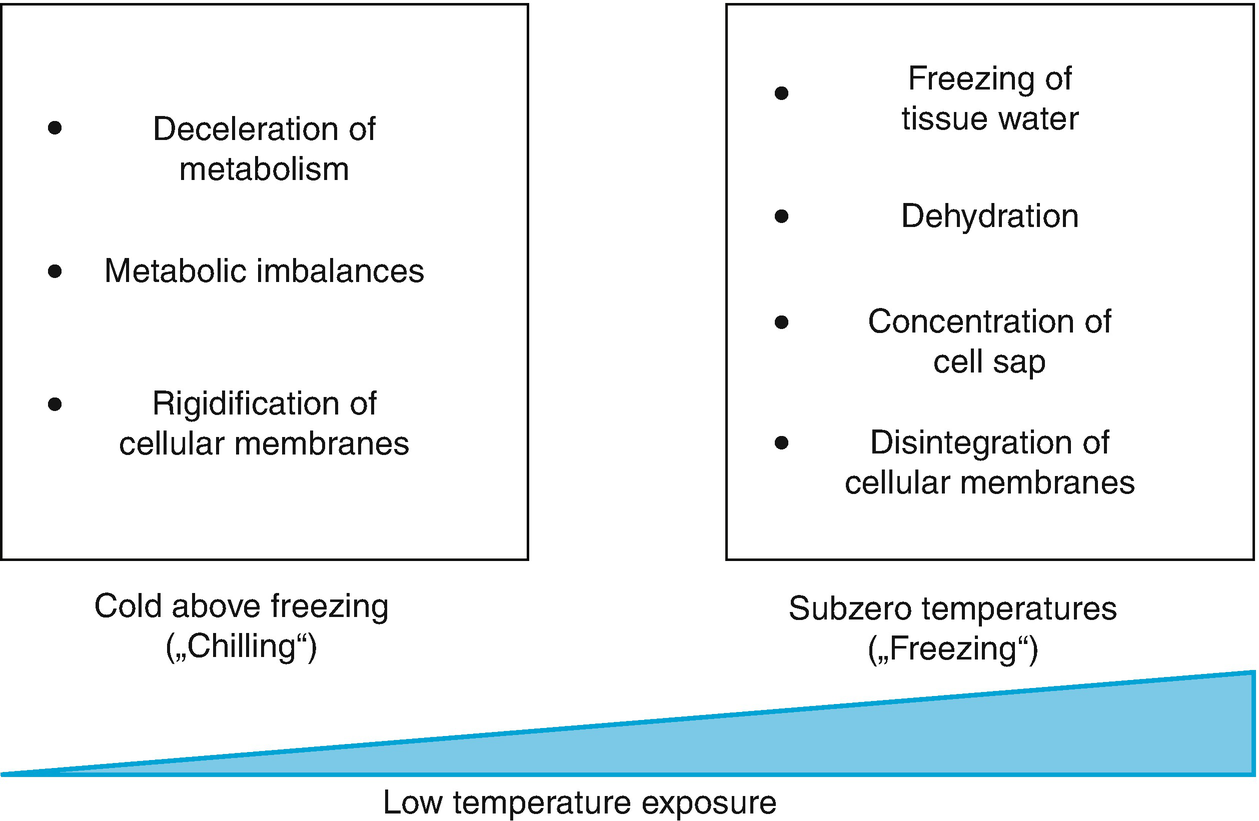
Stress from cold and frost at the cellular level. Low temperatures below the optimal range but above the melting point of water (= chilling) slow down enzymatic reactions. Because these are not equally affected by temperature changes (i.e. they have different Q 10 values), metabolic imbalances (Jones et al. 1998; Janská et al. 2010) occur. Cellular membranes rigidify as a result of reduced movement of lipids. A further decrease in temperature below the melting point (= freezing) can lead to the freezing of extracellular water. This causes dehydration because of a massive reduction in water availability owing to crystallisation. Cells lose water to the exterior and cell sap becomes more concentrated. Intracellular formation of ice crystals causes disintegration of cellular membranes and thereby ion leakage

Disintegration and reconstruction of a phospholipid bilayer by water removal (e.g. freeze dehydration) and rehydration. Phospholipids form lipid droplets or threads in the hexagonal II phase. (Modified from Crowe et al. (1983))
Box 4.1: The Physics of Freezing: Homogeneous and Heterogeneous Ice Nucleation

Thermograms of the freezing and thawing processes of pure water a and of an aqueous solution of a low molecular weight compound b. Δ denotes freezing point depression. FE freezing exotherm, FP freezing point, MP melting point, NT nucleation temperature, SC supercooling

Partial pressure of water vapour (in millibars) above ice and supercooled water
°C |
Vapour pressure of ice (mbar) |
Vapour pressure of liquid water (mbar) |
---|---|---|
0 |
6.1 |
6.1 |
−5 |
4.0 |
4.2 |
−10 |
2.6 |
2.9 |
−15 |
1.7 |
1.9 |
−20 |
1.0 |
1.3 |
−30 |
0.38 |
0.51 |




It follows that the freezing/melting point of a solution is lower than that of pure water (Fig. 4.6b) and that the depression (ΔTm) is proportional to the concentration of the solution, whereby the molar ΔTm = 1.86 °C; thus the actual ΔTm = 1.86 × n s (mole solute in 1 kg water (molality)).
This applies strictly to dilute (i.e. “ideal”) solutions. In concentrated solutions, interactions occur between the dissolved particles, resulting in an apparent reduction in the concentration. The factor α, by which the concentrations appears to decrease, is the activity factor.
The following considerations are important for the freeze dehydration of plant cells. Cooling of dilute solutions to sub-zero temperatures results first in freezing of pure water and an increase in the concentration of the cellular liquid. Separated by the cell wall, extracellular ice coexists with the liquid content of the cell, the proportions responding to the water potential of both compartments (within the cell, equilibrium of the water potentials of the organelles must also be assumed, otherwise considerable intracellular fluxes of water would occur between the organelles).
Box 4.2: How Much of the Tissue Water Can Freeze?

Freezing curve of frost-hardened ivy
(Hedera helix) leaves. The
curve shows the liquid water proportion (R L) of the total water
content (L 0)
as dependent on the frost temperature (t)4.9Upon ideal equilibrium freezing, a
rectangular hyperbola is obtained, which can be transformed into a
simple linear relationship (insert). The point at which this line
cuts the y axis is the
K point, indicating the
non-freezable portion of tissue water (Hansen and Beck 1988).
4.1.3 Variations in Temperature Range
A classification most commonly used in microbiology sorts organisms into three categories based on their temperature optima: psychrophiles, mesophiles and thermophiles. Psychrophiles are organisms adapted to cold habitats where water frequently changes from the liquid to the crystalline state. Most of them are unicellular snow and ice algae (e.g. Haematococcus pluvialis and various diatoms) or bacteria that prevent freezing of cellular water. At the other end of the scale are the thermophiles (and extremophiles) with optimal temperatures for growth between +80 °C and above 100 °C. They comprise certain unicellular algae such as Cyanidioschyzon merolae, cyanobacteria, bacteria and archaea, most of them living in hot springs, in geysers or on the black smokers of the deep sea. Most organisms, including practically all plants—in particular, the (homoiohydric) terrestrial and the marine species—belong to the mesophiles, which thrive at day temperatures between 15 °C and 25 °C and (in the case of terrestrial species) night temperatures about 10 K lower.

Damage to the African violet (Saintpaulia ionantha) by various chilling treatments. Below the threshold temperature of +8 °C, necrosis occurs. The strength of the chilling stress can be estimated as the product of cold (above the freezing point) multiplied by the duration of the exposure to the temperatures, as indicated in the figure. (Modified from Larcher (1994))

Differences in the cold sensitivity of the organs of a coffee tree. Visual damage after 3 days of continuous cooling to +1 °C is shown. Black denotes complete damage. Chl chlorotic (Larcher 2003)
The chilling sensitivity of the African violet has been used to demonstrate that the strength or quantity of stress is composed of its intensity and its duration (Fig. 4.8). Chilling damage, visible as necroses, starts at temperatures below 8 °C. Fifty per cent damage results from 6 h of exposure to +1 °C or maintenance at +5 °C for 50 h. In this case, the low rate of repair allows comparative quantification of stress damage.
The variation in the ability to tolerate temperature extremes is far less pronounced at the other end of the temperature scale. Critical temperatures causing heat damage barely vary between plants, and hardly any correlation with the climatic zone of origin is apparent (Tables 4.3 and 4.4).
Not all life processes of a plant have the same optimum temperature, because the individual developmental steps may take place in different ambient conditions. Winter cultivars of cereals, for instance, germinate at temperatures considerably lower than those required for growth (in spring and summer). Nevertheless, the rates of germination show typical temperature dependence with Q10 values between 1.5 and 2.5. In that context it is also important to mention that not all organs of a higher plant are similarly cold sensitive or tolerant. Those most sensitive are the roots and meristems of the shoots, while axial tissues and mature leaves are the most tolerant parts (Fig. 4.9). Also, certain stages in the development of a plant are particularly vulnerable—namely, seed germination and fruit ripening.
Maximum temperature resistance of microorganisms and poikilohydric seed plants in the moist turgescent state and in the dry rigid state caused by desiccation. (Modified from Larcher (2003))
Organisms/plant type |
Temperature at which cold damage occurs after at least 2 h (°C) |
Temperature at which heat damage occurs after 30 min (°C) |
||
---|---|---|---|---|
Moist |
Dry |
Moist |
Dry |
|
Bacterial spores |
Liquid nitrogen |
80 to 120 |
Up to 160 |
|
Fungi |
||||
Plant pathogenic fungi |
45 to 65 (70) |
|||
Saprophytic fungi |
0 to below −10 |
40 to 60 (80) |
75 to 100 |
|
Fungal fruiting bodies |
−5 to −10 (−30) |
|||
Fungal spores |
Liquid nitrogen |
50 to 60 (100) |
Above 100 |
|
Marine algae |
||||
Tropical sea algae |
+14 to +5 (−2) |
32 to 35 (40) |
||
Temperate sea algae (tidal zone) |
−8 to −40 |
30 to 35 |
||
Polar sea algae |
−10 to −60 |
(15) 20 to 28 |
||
Freshwater algae |
−5 to −20 (−30) |
35 to 45 (50) |
||
Airborne algae |
−10 to −30 |
Liquid nitrogen |
40 to 50 |
|
Lichens |
||||
From polar regions, high mountains, deserts |
−80 |
Liquid nitrogen |
||
From temperate climate zones |
−50 |
Liquid nitrogen |
33 to 45 |
70 to 100 |
Mosses |
||||
From humid tropics |
−1 to −7 |
|||
From temperate climates |
||||
From humid locations |
−5 to 15 |
40 to 45 |
||
From forest floors |
−15 to −25 |
40 to 50 |
80 to 95 |
|
Epiphytic and epipetric |
−15 to −35 |
Liquid nitrogen |
100 to 110 |
|
From polar regions |
−50 to −80 |
Liquid nitrogen |
||
Poikilohydric ferns |
−20 |
Liquid nitrogen |
47 to 50 |
60 to 100 |
Seed plants |
||||
Ramonda myconi |
−9 |
Liquid nitrogen |
48 |
56 |
Myrothamnus flabellifolia |
Liquid nitrogen |
80 |

Clinal variations in the freezing tolerance of Arabidopsis thaliana accessions. Survivorship is plotted against the January mean temperature at the site of origin for 24 A. thaliana accessions cooled to −10 °C for two consecutive nights. All plants were first cold acclimated for 7 days at 4 °C. The data are based on 20 replicates per accession (Zhen and Ungerer 2008)
Depending on the temperature climate of their habitat, plants must be adapted to and acclimate to not only the extremes of the temperature range (i.e. to seasonal variations) but also to smaller or greater diurnal temperature fluctuations. Considerable diurnal temperature oscillations are typical of deserts and the alpine and nival vegetation belts of high mountains, especially in the tropics. The air temperature during daytime may reach 20–30 °C, whereas the nocturnal temperature usually falls below the freezing point and the leaves may be stiffly frozen at the end of the night. While freezing of leaf tissue water is a process of several hours, thawing takes place within minutes once the tropical morning sun hits the leaves (Sect. 4.2.6). These plants have developed mechanisms to slow down freezing or even avoid it. They have to be frost tolerant all year round.
On a sunny day in temperate regions (e.g. in Central Europe) the surface of bare soil may become as hot as +50 °C, while the air temperature above the soil decreases rapidly with the distance from the soil surface. The gradient in the soil itself is even steeper. Reverse temperature gradients occur also during clear nights when no cloud cover mitigates radiation emission from horizontal and slightly inclined surfaces of the vegetation or from the soil. Young plants whose shoots or leaves are close to the ground are therefore exposed to a stronger temperature stress during both day and night than plants whose leaves are supported by a stem, which experience a considerably less challenging temperature microclimate (for details of the energy balance of leaves, Chap. 9).
Box 4.3: Measurement of Cold Hardiness and Damage by Cold and Freezing of Plant Tissue

Temperature course of a freeze–thaw cycle experiment. In order to determine the degree of cold hardiness or damage, the samples must be cooled to several defined final temperatures, where they remain for 2 h. Rewarming then occurs at the same rate as cooling. After rewarming, the sample is kept for 5 h at +5 °C and subsequently for 24 h or even longer at ambient temperature

Electrical conductivity assay. Damage to plant tissues leads to loss of selective membrane permeability—that is, inactivation of ion channels and transporters in plant membranes. Consequently, solutes leak out of the tissue. When, for example, excised leaf discs are floated in distilled water a, low molecular weight compounds diffuse into the medium, among them several ions. The increase in the conductivity of the water can be measured conductometrically. The more cells have been damaged, the higher the resulting conductivity is b
4.1.4 Strategies to Cope with Temperature Fluctuations and Temperature Extremes
Seasonally varying temperature extremes can be avoided through various escape strategies (Chap. 2). Annual plants can endure unfavourable conditions as dry seeds that are far less frost or heat sensitive. A new life cycle then starts with germination only after a cold winter or a hot, dry summer, depending on the habitat. Alternatives for perennial plants are the shedding of cold-sensitive organs such as the leaves or the overwintering only of the subterranean plant parts (rhizomes, bulbs) (Chap. 12). Tropical alpine plants have developed mechanisms of short-term freezing avoidance, as the daily periods of sub-zero temperatures are usually shorter than 12 h (Sect. 4.2.6).
Besides such escape strategies, every plant needs to be able to adjust metabolic fluxes and membrane fluidity to fluctuating temperatures and to protect cellular structures during short periods of temperature extremes. In the absence of homeostatic temperature regulation, most of the mechanisms involved operate at the cellular level and protect the tissues directly exposed to an unfavourable temperature.
Temperature tolerance of the leaves of vascular plants from various climate zones. (Modified from Larcher (2003))
Plant functional type |
Temperature at which cold damage occurs in a hardened state (°C) |
Temperature at which heat damage occurs during vegetative growth (°C) |
---|---|---|
Tropical plants |
||
Trees |
+5 to −2 |
45 to 50 |
Tropical alpine plants |
−5 to −15 (−20) |
About 45 |
Subtropical plants |
||
Evergreen woody plants |
−8 to −12 |
50 to 60 |
Palms |
−5 to −14 |
55 to 60 |
Succulents |
−5 to −10 (−15) |
58 to 67 |
C4 grasses |
−1 to −5 (−8) |
60 to 64 |
Annual desert grasses |
−6 to −10 |
50 to 55 |
Temperate plants |
||
Evergreen plants of mild winter |
−7 to −15 (−25) |
46 to 50 (55) |
Herbaceous plants |
−10 to −20 (−30) |
47 to 52 (sun), 40 to 45 (shade) |
Halophytes |
−10 to −20 |
|
Water plants |
−5 to −12 |
38 to 44 |
Homoiohydric ferns |
−10 to −40 |
46 to 48 |
Winter-cold areas |
||
Evergreen conifers |
−40 to −90 |
40 to 52 |
Boreal deciduous trees |
Liquid nitrogen (vegetative buds) |
42 to 45 |
Arctic alpine dwarfs shrubs |
−30 to −70 |
48 to 54 |
Acclimative changes in heat tolerance (= acquired thermotolerance), albeit not nearly as pronounced as cold hardening, are also well established. The underlying mechanisms are only partly specific to the stressor heat (Sect. 4.3); rather, they provide general stress tolerance. This is important, as heat stress (a) occurs in a temperature range of high metabolic activity and (b) is almost always accompanied by or associated with other stresses such as drought, high light intensity and generation of ROS.
4.2 Cold Acclimation and Freezing Tolerance
A complex array of cellular mechanisms (a cold tolerance syndrome) counteracts the negative consequences of exposure to low non-freezing temperatures and to temperatures below 0 °C. The efficiencies and relative contributions of different mechanisms vary between plant species, as well as depending on the mode of stress (e.g. acute versus chronic freezing stress). Also, the amount of direct evidence supporting the function of a particular mechanism for survival at low temperatures varies. Clearly of central importance is the adjustment of membrane fluidity through the modulation of lipid compositions. Furthermore, metabolic imbalances causing, for instance, the risk of photoinhibition need to be buffered. Various proteins and metabolites that protect cellular structures are synthesised during cold acclimation.
With respect to stress caused by sub-zero temperatures, mechanisms can be divided into tolerance and avoidance strategies—that is, those enabling plants to endure the formation of ice crystals versus those suppressing or even promoting the formation of ice crystals. Several reactions upon freeze dehydration resemble those observable during drought or salinity stress (Chaps. 6 and 7). At the molecular level, signal transduction networks activated by the three different types of stress target partly the same genes because some protective mechanisms are the same (Yamaguchi-Shinozaki and Shinozaki 2006; Huang et al. 2012) (Chap. 2). This explains the so-called cross-protection: exposure to drought improves cold tolerance, and vice versa. The same applies to osmotic strain caused by salt.

Mechanisms that allow plants to survive in habitats characterised by permanently, transitorily or seasonally occurring cold and frost
4.2.1 Adjustment of Membrane Fluidity


Fatty acid composition of membranes of Arabidopsis thaliana leaves grown at various temperatures from 17 °C to 36 °C. c + tr cis and trans isomers. (Modified from Falcone et al. (2004))
Membrane sterols play a special role, due to an amphipathic structure that differs from that of the glycerolipids. Since their hydrophobic moiety is much larger relative to the polar heads than in glycerolipids, they are able to diffuse rapidly horizontally as well as vertically (the so-called flip-flop mechanism). They serve as buffers for membrane fluidity. At low temperatures, they increase membrane fluidity by disrupting gelling of phospholipid domains and thus preventing the formation of semi-crystalline patches. At high temperatures, they decelerate the motion of the fatty acid tails and thus stiffen the membrane (Buchanan et al. 2015).

Chilling tolerance and photosynthetic performance of fatty acid desaturase–overexpressing transgenic tomato plants. a, b Chilling tolerance of non-acclimated plants a and acclimated plants b determined with the electrolyte leakage assay (Box 4.3). Green bars represent the untransformed wild type; other colours represent independent transgenic lines. Relative conductance was measured before cold treatment and after a 24-h recovery period subsequent to chilling in the dark for 3 days at 6 °C, 4 °C and 0 °C. c, d Photosynthetic performance determined by the chlorophyll a fluorescence assay (F v/F m; Chap. 3, Fig. 3.11) of wild-type and fatty acid desaturase–overexpressing tomato before cold treatment (25 °C) and after chilling, as described for a and b. All measurements were taken on the youngest fully expanded leaves. Acclimation was achieved by progressive cooling of the plants to a temperature of 10 °C. (Modified from Domínguez et al. (2010))
Adjustment of the lipid composition of membranes takes time, as degradation, synthesis and trafficking of lipids are required. Temperature changes during the course of a day/night cycle are probably too rapid, as changes in membrane composition usually take several days. Still, during a day/night cycle associated with a corresponding diurnal temperature cycle, the plasma membranes of Arabidopsis seedlings showed slightly lower fluidity during the cold phase. Whether this was due to a reorientation of the membrane domains (van Meer et al. 2008) or to small changes in lipid desaturation was not clear. The fluidity buffer function exerted by the membrane sterols may play an important role in compensating for diurnal fluidity fluctuations (Martinière et al. 2011).
Changes in the composition of the lipids of a defined biomembrane (the chloroplast envelope of spruce needles) during the course of frost hardening (“winter”) and de-hardening (“summer”) (Senser and Beck 1992)
Lipids |
Fatty acids (%) 16:0 + 18:0 + 18:1tr |
Fatty acids (%) 16:3 + 18:1 + 18:2 + 18:3 + 18:4 + 18: 2-hydroxy +20:3 |
---|---|---|
Phospholipids |
||
Summer |
55.4 |
41.2 |
Winter |
25.6 |
66.0 |
Galactolipids |
||
Summer |
33.4 |
64.4 |
Winter |
9.4 |
84.8 |
4.2.2 Prevention of Photoinhibition

Frost resistance and photosynthetic capacity (CO2 uptake at saturating light and 1% CO2 concentration) of one generation of spruce (Picea abies Karst.) needles measured under identical conditions over the course of 2 years. Note that the annual fluctuation in photosynthesis follows the dynamics of the chlorophyll content of the chloroplasts. Needles were taken from a 50-year-old spruce tree in the Botanical Garden in Munich. (After Senser and Beck (1979))
The limitation of photosynthesis does not necessarily result in a shortage of plant reserve material, as respiration is also markedly restricted at lower temperatures (Hansen and Beck 1994). Concomitantly with the slowdown in photosynthesis, frost hardening of evergreens is associated with a change in carbohydrate metabolism in favour of the production and accumulation of oligosaccharides instead of starch (Hansen et al. 1997).
4.2.3 Cryoprotective Proteins
In the course of cold acclimation, the proteome of plant cells undergoes pronounced changes. Many proteins increase in abundance upon exposure to low temperature. Collectively they are often referred to as cold-responsive proteins (COR proteins). Among them are isoforms of housekeeping enzymes with a lower temperature optimum, as well as enzymes involved in the biosynthesis of cryoprotectants (Sect. 4.2.4.1). The integrity and function of biomembranes have to be protected against damage resulting from freeze dehydration and the concomitant increase in the solute concentrations of the intracellular fluids. Such protection has been associated with the synthesis of dehydrins (DHNs) and dehydrin-like proteins (Late Embryogenesis Abundant (LEA) proteins and Responsive to ABA (RAB) proteins; for more details, Chap. 6). These proteins are all extremely hydrophilic glycine-rich proteins of a wide range of molecular weights. They lack enzymatic activity, are heat and acid stable and possess a high proportion of a random coil secondary structure, which binds water intramolecularly (Hanin et al. 2011). Interaction with biomembranes is enabled by a hydrophobic domain while the hydrophilic domain(s) face the cytosol. Attachment to the biomembranes displaces low molecular weight solutes, such as ions, from the membrane, thus avoiding changes in the membrane potential and disintegration or inactivation of ion pumps and transporters.
In unstressed plants, only low concentrations of dehydrins are present. Stress by drought, salt and cold leads to rapid up-regulation of gene expression and steady accumulation of dehydrins in all compartments of the cell. As for the majority of the COR proteins, formation of dehydrins can be triggered by abscisic acid (ABA) or can be activated independently of ABA. The promoter of the COR gene, RD29A (also known as COR78 or LTI178) contains cis-acting elements for ABA-independent signalling (cold response, osmotic stress) as well as for ABA-dependent (osmotic) signalling (Chaps. 2 and 6). Enhanced frost tolerance of A. thaliana was achieved by overexpression of RcDHN5 from Rhododendron catawbiense (Peng et al. 2008) and was attributed to maintenance of enzyme activity upon water deficiency. Nonetheless, the question as to whether particular dehydrins directly contribute to chilling and freezing tolerance remains unanswered.
Another type of proteins has been described as Cold Shock Domain Proteins (CSPs), suggesting cryoprotective properties (Sasaki et al. 2007). The typical Cold Shock Domain (CSD) contains a five-stranded β-barrel sheet with two consensus motifs, which bind to single-stranded DNA/RNA (Sasaki et al. 2013). The CSDs are found in bacteria, as well as in eukaryotes, and are considered RNA chaperones. Upon cold treatment, RNA forms unfavourable secondary structures that could interfere with RNA functions such as transcription and translation. CSPs are able to resolve these structures and restore RNA functionality. Although they are associated with cold adaptation, CSPs regulate many biological processes such as growth and flowering, thus exhibiting pleiotropic effects (Sasaki and Imai 2012).
4.2.4 Control of Ice Formation

Formation of intracellular ice in protoplasts from frost-sensitive a and frost-hardened b rye leaves as a function of the cooling rate and the minimum temperature (x axis). Protoplasts from frost-sensitive leaves do not deposit plasma membrane material on the outer surface upon shrinking by extracellular ice formation. Intracellular ice formation is lethal for the cells. (Modified from Dowgert and Steponkus (1983))
Whether crystallisation takes place inside or outside the cells depends on the cooling rate. When tissue is cooled slowly, water can move or leak out of the cells and crystallise outside the cell membranes in the extracellular spaces. This is a reversible process following the equilibrium between the water potentials of ice and of the cellular solutions. The water potential of ice is more negative than that of supercooled pure water (Box 4.1). The lower the sub-zero temperature is, the more negative the water potential of ice is and the more cellular water crystallises in the intercellular space. At a high cooling rate, there is not sufficient time for water export, and freezing takes place intracellularly, thereby killing the cells. In that case, ice crystals can grow through plasmodesmata and pits from cell to cell and rapidly expand to wider areas of the tissue.
For crystallisation, the liquid accumulating in the apoplastic space requires a nucleation trigger. Ice spreading from nearby xylem vessels could play such a role. In frost-sensitive dicots, ice spreads from only one nucleation event rapidly through the entire plant, while in graminoids, because of the polystele, each grass leaf requires separate nucleation. Thus, the shoot structure and vascular system are important for the spreading of ice and freezing damage (Hacker and Neuner 2007, 2008). Otherwise, so-called ice nucleation–active bacteria (INA bacteria; Box 4.4) in the substomatal cavity or on the surface of a leaf could trigger nucleation. With potato and cauliflower, it has been shown that even water droplets on a leaf or a flower bud surface can catalyse ice formation inside the plant, and it takes only a few minutes for the entire plant to be frozen (Fuller and Wisniewski 1998). Ice nucleation activity has recently been reported in plant cell walls of bark tissue from blueberry stems, with outstanding activity (ice nucleation at −1.0 °C). This activity showed seasonal changes, peaking in November shortly before the onset of frost (Kishimoto et al. 2014). The mechanism underlying this INA principle is not yet known.
Box 4.4: Ice Nucleation Proteins
Ice nucleation activity of bacterial cultures (Maki et al. 1974)
Species |
Ice nucleation temperature (°C) |
|
---|---|---|
T 1 |
T 90 |
|
Pseudomonas syringae C9 |
−2.9 |
−3.5 |
Pseudomonas syringae wt |
−3.2 |
−3.9 |
Pseudomonas aeruginosa |
−7.5 |
−17.8 |
Staphylococcus epidermidis |
−6.9 |
−19.5 |
Escherichia coli |
−8.3 |
−17.1 |
Proteus mirabilis |
−8.0 |
−19.4 |
Bacillus subtilis |
−10.6 |
−18.0 |
Pure culture medium |
−9.2 |
−17.0 |

Blueprints of the ice nucleation protein (INP) from Pseudomonas syringae a and INP-F from the fungus Fusarium acuminatum b. Triangles represent the repetitive motif. The N-terminal domain (about 15%) of the Pseudomonas INP consists of three or four potential transmembrane spans; the C-terminal tail makes up 4% of the protein (Shimazu et al. 2003). The smaller number of the IN motifs in the fungal protein result in lower ice nucleation activity: P. syringae: −2 °C, F. acuminatum: −5 °C. (Lagzian et al. 2014)
Analysis of bacterial ice nucleators (INPs) from Pseudomonas syringae. (Hew and Yang 1992)
Nucleation temperature (°C) |
Estimated molecular mass (kDa) |
Number of INPs required for nucleation |
---|---|---|
−12 to −13 |
150 |
1 |
−3 |
870 |
60 |
−2 |
19,800 |
132 |
−1 |
83,700 |
558 |
As mentioned above, ice seeding activity has been observed with plant cell walls from blueberry stems and also with cell walls from rye leaves (Brush et al. 1994). Apart from a general chemical composition (proteins, carbohydrates and phospholipids (Gusta et al. 2004)), the mode of function and mechanism of plant ice nucleators are not yet known.
Antagonists (mutants) of ice nucleation–active (INA) bacteria inhibiting noxious ice formation during moderate frost. (After Lindow (1982))
Bacterial culture |
Damage (% change in leaf colour after 1 week) |
---|---|
Control (Pseudomonas syringae) |
95 |
Mutant A 510 |
12 |
Mutant A 509 |
18 |
Mutant A 507 |
27 |
Mutant A 506 |
33 |
Mutant A 508 |
51 |
INA bacteria have found several technical and biotechnical applications: artificial snow making, cloud seeding and rain making in warmer regions, and use as part of a fusion protein of the N-terminus with a protein of interest (e.g. an enzyme (Gao et al. 2014)) or binding of cancer cells by an antibody or antibody mimetic protein displayed on the surface of engineered Escherichia coli cells (Zahnd et al. 2007).
4.2.4.1 Osmotic Adjustments
For the sake of survival during freezing, ice formation should take place outside the cell walls in the intercellular spaces and should be suppressed intracellularly by furnishing of the cells with compatible solutes, which allow them to supercool, thereby preserving their cell plasma in the liquid state. Both strategies entail benefits and stresses or risks. Extracellular freezing of cellular water results in cellular desiccation proportional to the frost temperature. At the same time, intracellular solutions concentrate and may exert ionic stress on biomembranes and protein complexes. Accumulation of compatible solutes counteracts problems caused by freeze desiccation, but this mechanism carries a high risk as ice formation by overstretching the capacity for supercooling leads to instantaneous death, as there is no time for water export. For both mechanisms, stress increases with the strength of the frost. Possible damage is gradual upon extracellular freezing but is sudden and absolutely detrimental upon intracellular ice formation.
Accordingly, frost hardening of plants is regularly associated with the accumulation of compatible solutes (also termed osmolytes or cryoprotectants) in the cells, predominantly in the vacuoles which, however, have to equilibrate their osmotic potential with the cytoplasm and other cellular organelles. These low molecular weight solutes are carbohydrates, amino acids (e.g. proline), polyamines and many more. Among the carbohydrates that accumulate during frost hardening, sucrose and its galactosides raffinose and stachyose are prominent. Also, polyols, as the reduced forms of monosaccharides, are frequently found. For winter and summer cereals, for instance, a strong correlation between freezing tolerance and the sugar accumulation rate was determined.
Compatible solutes, in addition to their colligative effects, are known also as soft ROS detoxifiers (e.g. mannitol) (Tarczynski et al. 1993). Upon extracellular freezing of cell water, these non-charged but weakly polar (like water) compounds “dilute” the ionic charge at the membrane surfaces (which results from the concentration of cellular ionic compounds) and thus stabilise the bilayer structure.
Their colligative (physicochemical) effect on the freezing point of the cellular liquids comes into play when freeze dehydration progresses. For example, the cell sap of frost-hardened spruce needles is only 1.5 osmolar, corresponding to a freezing point depression of 2.8 K. This is physiologically insignificant because ice nucleation requires stronger supercooling than −2.8 °C. Upon extracellular freezing of cellular water the concentration of the cryoprotectants increases significantly, and with a 10% remaining liquid volume, the freezing point depression attains 28.5 K, which could prevent freezing of the residual liquid water.

Frost protection in the alpine Primula clusiana Tausch a. b The branched-chain polyol hamamelitol. c The disaccharide clusianose (d-galactosyl-hamamelitol). d Annual course of the concentration of carbohydrates in the leaves of that primrose. Accumulation of carbohydrates can also be induced by cooling of the plants in summer for 1 week at 5 °C. (Photo: E. Beck)
Besides the formation of cryoprotectants, the release of water from the cell into the apoplast can further reduce the probability of intracellular freezing, albeit at the cost of potentially stronger ionic stress for the cell. Membranes of frost-hardened cells allow water to exit from the cell into the apoplast more easily than frost-sensitive membranes do. This has been demonstrated with protoplasts isolated from frost-sensitive and frost-hardened rye leaves (Fig. 4.17). The mechanisms behind this facilitated efflux are not known. The simplest explanation assumes cold inhibition of ion pumps and H+-ATPases, leaking of low molecular weight solutes and passive water efflux, following the solutes.
4.2.4.2 Antifreeze Proteins
In particular, unicellular organisms such as bacteria are endangered by intracellular crystallisation of water. Three strategies have been recognised in frost-tolerant organisms including prokaryotes, lower and higher plants, and special groups of animals—for example, insects or inhabitants of the Arctic and Antarctic Oceans. Apart from the accumulation of compatible solutes and the activation of their biosynthetic machinery, three functional types of proteins can be differentiated in relation to the formation of ice crystals at the cellular level: proteins that promote ice formation (ice nucleation proteins (INPs)), proteins that inhibit ice nucleation (anti-nucleating proteins (ANPs)) and proteins that control the growth and recrystallisation of ice crystals (the classical antifreeze proteins (AFPs)). ANPs and AFPs are often considered one group of proteins because there is some evidence that AFPs might also bind to nucleation-promoting structures (Griffith and Yaish 2004). Up to now, INPs have been known only from prokaryotes—mainly gram-negative bacteria (Box 4.4)—while ANPs and AFPs are also known from plants.
Lowering of the freezing point by antifreeze proteins, as measured by thermal hysteresis
Organism |
Tissue |
Lowering of freezing point, thermal hysteresis (°C) |
---|---|---|
Bony fish |
Muscle |
0.7–1.5 |
Insects |
Digestive tract, muscle |
3–6 |
Bacteria |
– |
0.3–0.35 |
Fungi |
Fruiting body |
0.3–0.35 |
Mosses |
Whole plant |
0.3–0.68 |
Horsetails (Equisetum hyemale) |
Rhizome |
0.2 |
Ferns |
Shoot |
0.25 |
Winter wheat |
Leaves |
0.2 |
Carrot |
Root |
0.4 |
Carrot |
Shoot |
0.15 |
Poplar |
Twig |
0.22 |

a Hexagonal ice crystal (blue) that forms in the presence of antifreeze proteins (AFPs) showing the α (basal) and c (prism) axes and the modes of interaction with AFPs. Binding of AFPs (brown symbols) to the “vertical” prism plane inhibits growth of the crystal’s basal plane (width), while binding to the basal plane prevents growth along the c axis (crystal length). b In pure water, ice grows as a round and flat crystal. c In a dilute solution, most types of AFP bind to the prism face of ice creating the hexagonal crystals. d Adsorption of AFPs to the prism face inhibits the binding of additional water molecules, making it energetically favourable for water to bind to the basal plane so that the crystal grows along the c axis (towards the viewer). e At high concentrations of AFPs, the ice crystals form bipyramids a, which are hexagonal in cross-section. f When the temperature is cooled and warmed in slow cycles, it is possible to see ridges on the surface of the ice crystal where the AFPs have bound. Scale bar = 10 μm. (a From Lorv et al. (2014); b–f from Griffith and Yaish (2004))
The efficacy of AFPs in lowering the freezing point differs greatly between organisms. Plant AFPs, although not very effective in lowering the freezing temperature (less than 1 K; Table 4.9) are very efficient in inhibiting recrystallisation of ice nuclei. Because of multiple ice-binding domains of individual plant AFPs or AFP oligomers, the same protein can bind simultaneously to different planes of the ice crystals (Griffith and Yaish 2004). Intracellular AFPs, when binding to ice nucleators, can prevent intracellular ice formation. Furthermore, they can be secreted into the apoplast, where they interfere with growth and recrystallisation of extracellular ice. Thus, they have a dual task.
Amino acid sequences of plant antifreeze proteins do not establish them as a distinct protein family. Instead, AFPs are highly variable and display similarities to a wide range of proteins. Some AFPs—in particular, those from cereals—are highly homologous to pathogenesis-related (PR) proteins produced upon pathogen attack. They include chitinases, β-1,3-glucanases, polygalacturonase inhibitors, and osmotin- and thaumatin-like enzymes (Griffith and Yaish 2004; Gupta and Deswal 2014)—that is, enzymes that degrade fungal cell walls and inhibit fungal enzymes. In cold-acclimated winter rye, AFPs exhibit both antifungal and antifreeze activity. In contrast, PR proteins induced by pathogens at room temperature in non-acclimated plants lack antifreeze activity. The dual function of the PR-AFPs has been interpreted with respect to resistance against low-temperature pathogens such as snow mould. The excreted PR-AFPs form hetero-oligomers that inhibit growth of such fungal pathogens (Hiilovaara-Teijo et al. 1999).
4.2.4.3 Supercooling
Supercooling refers to the phenomenon where aqueous solutions remain in the liquid state even below the melting point (Box 4.1). Freezing avoidance by supercooling preserves the water relations of the turgescent tissue and prevents considerable export and import of water upon extracellular freezing. In woody tissues of boreal plants, water can supercool to the temperature of homogeneous ice nucleation. The classical example of deep or extreme supercooling is the xylem of dogwood (Cornus sericea, syn. Cornus stolonifera) (Byard et al. 2010). Several woody species have been shown to exhibit the phenomenon of deep supercooling, while others of similar geographical distribution do not (e.g. Acer supercools, whereas the majority of the investigated Betula species freeze) (Byard et al. 2010). Anti-nucleating substances such as flavonoids and hydrolysable tannins have been proposed to contribute to deep supercooling (Wisniewski et al. 2014). However, at present the mechanisms enabling deep supercooling are not known: “The properties of a cell, tissue or organ that allow it to deep supercool remain enigmatic despite the widespread prevalence of this ability in many plant species” Gusta and Wisniewski 2013 (see also Kasuga et al. 2008; Wang et al. 2013).
4.2.5 Signalling Networks Involved in Cold Acclimation
Drought, high salinity and freezing stress all pose osmotic challenges for plants. Accordingly, there is considerable overlap in the molecular responses of cells to these different stress conditions (Chap. 2). When one is judging by the hundreds to thousands of changes elicited in the A. thaliana transcriptome, however, there are also highly complex responses specific to cold acclimation.
A large number of COR genes belong to the CBF (for C-REPEAT BINDING FACTOR) regulon—that is, they are activated under the control of CBF transcription factors (also termed DRE-Binding proteins (DREB)) (Yamaguchi-Shinozaki and Shinozaki 2006). The promoters of these COR genes, which encode many of the proteins discussed above (i.e. dehydrins, antifreeze proteins, ROS-detoxifying enzymes, fatty acid desaturases, lipid transfer proteins, sugar and proline transporters, osmolyte producing enzymes) share a 5-bp consensus sequence (CCGAC), the so-called Dehydration Responsive Element (DRE), which is known also as Low Temperature Responsive Element (LTRE) or C-Repeat (CRT). Binding of CBFs rapidly activates transcription of COR genes.

Effect of CBF overexpression on the cold sensitivity of Arabidopsis thaliana. Wild-type plants a require several days of acclimation at low non-freezing temperatures to withstand freezing temperatures c, while plants overexpressing CBF genes survive transfer from warm temperatures directly to sub-zero temperatures b. Note the smaller size of the CBF-overexpressing plants in b. (Modified from Jaglo-Ottesen et al. (1998))

Cold acclimation pathway. Model for signalling leading from cold to the expression of COR genes regulated by CBF transcription factors in Arabidopsis. Transcription factors are represented by solid ellipsoids; other proteins are represented by circles. Red symbolises Ca-dependent processes, green symbolises processes controlled by MAP kinase cascades. S sumoylation, U ubiquitination, CaM calmodulin. (Modified from Knight and Knight (2012))
Events upstream from ICE1 are less well defined. The primary sensor of cold temperature is still unknown (Sect. 4.4). What is clearly established is a rapid increase in cytosolic Ca 2+ levels upon transfer of plants to cold conditions, which activates proteins that inhibit ICE1 degradation and activate ICE1 sumoylation. In addition, Ca2+ influences CBF expression via a calmodulin-binding transcription factor (CAMTA). The same regulatory steps leading to enhanced ICE1 activity are triggered by a cold-responsive Ca2+-independent MAP kinase phosphorylation cascade.
Box 4.5: The Role of the CBF Regulon in Cold Tolerance and Cold Acclimation

Correlation between habitat temperature, acclimated freezing tolerance a and gene expression b in particular, of the CBF regulon c–e of Arabidopsis thaliana accessions from different climates. The temperature data for the accessions are the average minimum temperature during January for winter annuals and April for summer annuals, obtained from the nearest weather station. Freezing tolerance is given as the temperature at which 50% electrolyte leakage occurred from detached leaves (LT50) of plants. For cold acclimation the plants were subjected to a temperature of 4 °C for 14 days. The data are means from 4–6 independent experiments. The acclimation capacity b was measured as the difference between the non-acclimated and acclimated LT50 of detached leaves. It correlates nearly linearly with the extent of changes in gene expression. Changes were classified as significant when the gene-wise false discovery rate–corrected F test and t test p values were both less than 0.05. c–e Expression of the CBF transcription factor genes CBF1–3 in cold-acclimated plants. Scatterplots of GCRMA (guanine cytosine robust multi-array analysis) on a log2 scale, where a doubling of expression intensity corresponds to a unit of 1. Expression estimates are shown against the LT50 of detached leaves from the nine accessions after 14-day cold acclimation. The data are means ± standard errors (SEs) from three independent experiments (Hannah et al. 2006)
4.2.6 Freezing Avoidance and Freezing Tolerance in Tropical High Mountain Plants

Temperature courses in a tropical “Frostwechselklima” recorded on Mt Kenya at 4200 m altitude during the dry season. The temperature sensor was about 20 cm above the soil surface

Young specimens of the giant rosette plant Senecio (Dendrosenecio) keniodendron in the Afro-alpine zone of Mt Kenya (at 3500–4800 m). These plants carry a single terminal leaf rosette of up to 1 m diameter with around 100 big leaves surrounding the terminal leaf bud (dark blue in the sketch) in the centre of the rosette. At least the first half of the leaf development takes place in that cone-shaped leaf bud, from which the leaves separate after greening. After senescence, the dead, now outer leaves of the rosette remain attached to the stem and the resulting leaf cloak of shrivelled leaves delays nocturnal cooling of the stem until the tropical sun warms it up again. Maintenance of the stem temperature well above the freezing point is important, as a large share of the water required by the leaves is supplied by the living pith cells, while the amount of water delivered by the extremely narrow vessels of the weakly developed xylem is inferior. Water transport via living cells depends strongly on the temperature. A similar “strategy” is known from the corresponding plant life form, Espeletia, in the tropical Andes, where the “cloak” of dead leaves has inspired the plant’s popular name, frailejones (“thick monks”). Interestingly, the cloak is densely rooted by adventitious roots from the stem, which absorb water and nutrients from the decaying leaf material. (Beck 1994b)
4.3 Heat Stress
Heat is understood here as the upper temperature range in which active life is possible (Fig. 4.1). In this range, stress increases with increasing temperature, and organisms (in particular, plants) that cannot escape the heat stress respond with a diverse set of reactions. Not all of them are specific to heat; they can also be observed under drought, salt and heavy metal strain—that is, whenever proteins are denatured. Nevertheless the heat stress response in plants has distinctive features and is very complex. In the tolerable temperature range it appears to follow the stress dose rule (the product of the severity of stress and exposure time: ΔT × t) (Nover and Höhfeld 1996). In mesophilic plants a slight heat stress response can be observed beyond 35 °C. Above 40 °C, strong reactions take place. Such temperatures can be reached in many plant habitats. On a sunny day in Central Europe, for instance, topsoil temperatures can transitorily exceed 50 °C, which can severely damage or even kill seedlings that have just emerged from the soil surface. Also, solar irradiation can cause very rapid increases in temperature by easily 20 °C within minutes (McClung and Davis 2010).

Heat damage on a leaf of Cannabis sativa, resulting in collapsed leaf patches. The pale colour indicates disintegration of the chloroplasts and photobleaching of chlorophyll liberated from their protein environment in the thylakoids. (Source: http://forum.sensiseeds.com/images/plant_problems/heat_stress_rd_c2727.html)
When one is considering the exposure of a plant to potentially dangerous elevated temperatures, heat shock should be differentiated from heat stress. A heat shock (even if it is not injurious) refers to a sudden and short-term exposure that triggers a transient short-term response, while heat stress refers to a long-term exposure to elevated and potentially damaging temperatures. Both heat shock and heat stress can lead to the so-called acquired thermotolerance (Sect. 4.3.2)—that is, the ability to withstand higher temperatures because of acclimation. The major difference at the cellular level is the rapid deceleration of housekeeping metabolism (apparent in the down-regulation of housekeeping genes) and the subsequent revival of this metabolism after a return to the normal temperature in the heat shock reaction, while in long-term heat stress exposure the housekeeping metabolism has to maintain its function at the elevated temperature. Both sets of reactions, however, depend on the strongly enhanced expression of genes encoding transcription factors (heat shock factors) and protective proteins (heat shock proteins), many of which serve essential cellular functions also at optimal temperature.
Effects of elevated temperatures on growth, tuber formation and starch content of a heat-tolerant potato (Solanum tuberosum) variety (“Norchip”) and a heat-sensitive variety (“Up-to-date”) (Lafta and Lorenzen 1995)
Cultivar |
Temperature (°C) |
Shoot (g/plant) |
Tuber (g/plant) |
Total weight (g/plant) |
Plant height (cm) |
Starch content (mg/g dry weight) |
---|---|---|---|---|---|---|
Norchip |
19/17 |
244 ± 6 |
134 ± 3 |
378 ± 4 |
35 ± 1 |
290 ± 9 |
31/29 |
253 ± 4 |
5 ± 3 |
258 ± 4 |
45 ± 2 |
129 ± 2 |
|
Up-to-date |
19/17 |
197 ± 17 |
131 ± 14 |
328 ± 13 |
42 ± 1 |
24 ± 14 |
31/29 |
96 ± 2 |
0.6 ± 0.6 |
96 ± 3 |
57 ± 0.5 |
99 ± 12 |
Activities of the enzymes starch synthase and ADP-glucose pyrophosphorylase (AGPase) in the potato tubers of “Norchip” and “Up-to-date” after 2 weeks of exposure to an elevated temperature regime (from Lafta and Lorenzen (1995)
Cultivar |
Temperature (°C) |
Sucrose synthase (mg sucrose/g × h) |
AGPase (μmol ADP-glucose/g × min) |
---|---|---|---|
Norchip |
19/17 |
33.4 ± 11.9 |
1.2 ± 0.11 |
31/27 |
13.7 ± 3.8 |
0.93 ± 0.05 |
|
Up-to-date |
19/17 |
15.0 ± 4.2 |
1.17 ± 0.12 |
31/27 |
4.2 ± 2.3 |
0.84 ± 0.08 |

Effects of heat on plants and their reactions to it
4.3.1 Heat Stress Avoidance
The timing of key developmental steps such as germination and sexual reproduction can help plants reduce the probability of heat exposure and damage (Chap. 2). Besides that, not many different mechanisms of heat stress avoidance have been demonstrated. At the morphological level, positioning of the leaves in a tree crown or canopy and the leaf angle may reduce heating by sunrays. Segmentation of large leaf areas into narrower parts can have a similar effect. Transpiration cooling (Chap. 10) appears to be an effective mechanism. However, heat frequently coincides with low water availability, and then the necessary closure of stomata greatly reduces the cooling potential. More details about heat avoidance are presented in Chap. 9. Generally, however, because of the extreme fluctuations in temperature that can occur daily or seasonally and at the micro-scale (Sect. 4.1), it is in most habitats virtually impossible for terrestrial plants, as sessile organisms, to avoid heat stress altogether. Accordingly, all plants are capable of mounting a response to heat stress (Vierling 1991).
4.3.2 Acquired Thermotolerance

Effect of hardening on the survival of heat-stressed Arabidopsis thaliana seedlings. a Protocol of the hardening experiment. Gradual hardening was accomplished by slow elevation of the ambient temperature from 22 °C to the heat shock temperature of 45 °C. Stepwise hardening was performed by application of a moderate heat shock (38 °C for 90 min) 2 h prior to the actual heat shock. No hardening treatment was applied in the control set of seedlings. Samples were returned to the ambient temperature and analysed for survival after 2½ days b. (c) Quantitative analysis of the acclimation effect. (Modified from Larkindale and Vierling (2008))

Acquisition of thermotolerance in fir needles after heat stress treatment. Damage and hardening were assessed by measurement of the photosynthetic activity of the needles. (1) Recovery and hardening phase after the first heat stress. (2) After the second heat treatment (44 °C for 30 min). The extent of damage and the duration of the recovery phase are clearly reduced after the second stress (Larcher (1987))
The most important mechanism underlying acquired thermotolerance is the massive production of heat shock proteins (HSPs) (Sect. 4.3.3.1) (Vierling 1991). Regardless of the optimal growing temperature, this response is highly conserved not only among plants but also among prokaryotes, animals and fungi. According to recent genome-wide analyses in A. thaliana, however, not only the expression level of HSP genes changes. Up to 2% of all genes are affected in their activity (Kotak et al. 2007). For many of them the contribution to thermotolerance is functionally not understood. What is evident is an up-regulation of anti-oxidative defences to counteract the increased production of ROS under heat stress. Documented is also the accumulation of so-called chemical chaperones, which can increase the heat resistance of cells and shift the onset of the heat stress response to higher temperatures. These chemical chaperones are compatible solutes such as glycerol, proline and betaines (Chap. 6). They stabilise the folding and structure of mature proteins, and they can buffer the cellular redox potential. Another critical response is the modulation of membrane lipids towards a higher saturation level of fatty acids. Some of the reactions may initiate signalling cascades that result in the induction of HSPs. For instance, the dehydration-responsive transcription factor DREB2A (Chap. 6) regulates the heat shock factor Hsf3A (Sect. 4.3.3.2 and Box 4.7). ABA concentrations rise in heat-stressed tissues.
4.3.3 The Heat Shock Response
Heat stress causes the unfolding (denaturation) of proteins (Sect. 4.3.3) (Qu et al. 2013). In eukaryotic cells this triggers a reaction sequence termed the unfolded protein response (UPR). Stress-induced accumulation of unfolded proteins activates transcription factors (heat shock transcription factors (HSFs)), which then bind to specific cis elements (heat shock elements (HSEs)) in the promoters of genes encoding heat shock proteins (HSPs).
4.3.3.1 Heat Shock Proteins
HSPs are named after their enhanced production upon heat treatment. However, they are essential for prokaryotic as well as eukaryotic cells, both in the absence of stress and for heat stress survival. HSPs act as chaperones required for correct folding of (unglycosylated) nascent proteins upon release from the ribosome or after stress-induced misfolding, unfolding or aggregation of unfolded proteins (Box 4.6). This essentiality is the reason why the contribution of HSPs to heat stress survival—while not in doubt—cannot be accurately assessed genetically. Loss-of-function mutants are not viable.
HSPs are constitutively expressed and involved in protein and membrane stabilisation, in protein assembly into functional complexes, in intracellular transport of protein precursors to their target organelles and as part of cellular signalling processes. In plants they occur in plastids, mitochondria, peroxisomes, the nucleus, the cytosol and the ER. However, not all chaperones occur in all of these plant cell organelles. Prokaryotic HSPs such as HSP60 are known only from mitochondria and chloroplasts—plant organelles of prokaryotic origin. HSPs are classified by their molecular weight (Wang et al. 2004) and only in the bacterial (Escherichia coli) system do they have specific designations (in brackets). The most important classes are HSP100 (Clp), HSP90, HSP70 (DnaK), HSP60 (GroEL, the so-called GroE chaperonins) and the small HSPs (sHSPs): the HSP40 (DnaJ), HSP10 (GroES) and HSP23 (GroE) families. HSPs can cooperate with co-chaperones (e.g. HSP70 with HSP40 and HSP23).
Chaperones, particularly of the HSP70 type, are also involved in the removal of irreversibly denatured proteins. After a strong heat stress, some unfolded proteins cannot be repaired anymore. They are irreversibly denatured and tend to form—or have already formed—aggregates via their exposed hydrophobic domains. Such proteins have to be removed from the cell. They are tagged for degradation in the 26S proteasome by the attachment of several units of the small protein ubiquitin (poly-ubiquitination). Targeting to the proteasome where the adenosine triphosphate (ATP)-consuming degradation takes place is then dependent on HSP activity.
Box 4.6: Chaperones and Chaperonins Repair Misfolded Proteins

Model of the mechanism of action of heat shock protein 70 (HSP70) chaperones in protein rescue. (Modified from Doyle et al. (2013))
The prokaryotic chaperonin GroEL/GroES cycle operates in the plastids and mitochondria of a plant cell in a mechanism that resembles that of the HSP70 system but also shows some differences, as the GroEL forms a back-to-back double chamber, with each chamber consisting of seven GroEL subunits, which all show ATPase activity. Both chambers work alternately. GroES is the lid protein and DnaJ replaces the J-domain protein. One GroEl cycle consumes seven ATPs. Step 3, as the rate-limiting step, requires about 10 s.
4.3.3.2 Heat Stress Transcription Factors
Transcriptional activation of HSP genes is dependent on heat stress transcription factors (Hsfs). Hsfs have a modular structure (Box 4.7) which, irrespective of variations in size, is conserved in the entire eukaryotic kingdom (Scharf et al. 2012). The mode of interaction with the promoters is equally conserved. Plant genomes are particularly rich in Hsfs: in A. thaliana, 21 Hsf genes are known; in soybean there are 52 such genes. According to similarities and differences in their sequences, plant Hsfs have been classified into HsfA, HsfB and HsfC, each class consisting of a number of members (e.g. HsfA1–A8). Further grouping is indicated by lower-case letters (e.g. HsfB4a–h). In comparison with plants the diversity of Hsfs in other organisms is smaller.

Regulation of the heat stress response in tomato. a The unstressed state. b Acute heat stress; free Hsfs oligomerise and bind to the heat stress elements of the promoters of the HSPs. More HSPs are produced, as well as more HsfA2, the key regulator in the plant heat stress response. c Prolonged heat stress leads to acquired thermotolerance; Hsfs A1 and A2, together with other general transcription factors (GTFs), form a superactivator for the expression of more HSPs and the formation of heat stress granula (HSG). d Recovery from heat shock and enhanced thermotolerance; expression of HSP genes is strongly attenuated and the Hsfs are either degraded in the proteasome or conserved by binding to HSPs. (Modified from Scharf et al. (2012))

Domains of the tomato HsfA2. (Modified from Scharf et al. (2012))
Upon abatement (Fig. 4.31d) of the heat stress, the heat stress response is switched off. HsfA2 is inactivated by association with small HSPs (e.g. HSP17-CII and HSP70). This complex accumulates in the so-called heat stress granula (HSGs; Sect. 4.3.3.3). Release of HsfA2 from these granula requires another small heat stress protein, HSP17C-1, which is important for long-term heat stress. Prolonged heat stress results in high levels of heat stress proteins and of HsfA2. This is the state of acquired thermotolerance. Fading of the heat stress releases HSPs because of the decreasing level of misfolded proteins. An increase in the concentrations of free HSPs leads to monomerisation and thus inactivation of the Hsfs because of their reassociation with the HSPs. HsfA1 binds again to HSPs 70 and 90, and HsfB1 binds accordingly to HSP90. This complex can elicit degradation of HsfB1. Furthermore, it can interact with transcription factors of housekeeping genes and reactivate their expression. HSP90 interacts with HSGs, whereupon HsfA2 is released and degraded.
The interaction between heat shock factors and heat shock proteins exerts effective control over the up-regulation of HSP synthesis. The presence of more denatured proteins than under non-stressed conditions triggers the interaction of Hsfs with HSP gene promoters, and a decline in the number of denatured proteins gradually tunes out the extra HSP synthesis. Nonetheless, other factors are known to contribute to the activation of the heat shock/heat stress response (Kotak et al. 2007; Saidi et al. 2011). These include ROS and the plant hormones ABA, ethylene and salicylic acid. A change in the ROS homeostasis resulting from ROS production and detoxification appears to be a signal. Several mechanisms of ROS sensing have been proposed—for example, unidentified receptor proteins and redox-sensitive transcription factors (Chap. 2), including heat shock transcription factors (Mittler et al. 2004). However, neither ROS signalling under heat stress nor the roles of signalling molecules are understood nearly as well as the Hsf-dependent activation of HSP synthesis triggered by protein denaturation.

Activation of the bZIP28 transcription factor by stress, such as heat stress. BiP binding protein, a member of the HSP70 family. (Modified from Srivastava et al. (2014))
Box 4.7: The Structure of Heat Stress Transcriptions Factors
In all investigated heat stress transcription factors (Hsfs), the DNA-binding domain is close to the N-terminus (Fig. 4.32). The hydrophobic core of that domain of about 100 amino acids specifies the selectivity of the interaction with the repetitive heat shock elements (HSEs) on the promoters of the heat shock genes. Since usually more than two HSEs are required for a proper heat stress response, oligomerisation of the Hsfs is also necessary for promoter activation.
Downstream from the DNA-binding domain is the Hsf oligomerisation domain (OD). Depending on the structure of this domain, homo-oligomerisation—as well as hetero-oligomerisation—is possible. The classification of HsfA, B or C results from sequence differences of the OD, which is therefore also termed the HR-A/B region. Clusters of basic amino acids downstream from the OD represent the nuclear localisation signal (NLS), which mediates import into the nucleus. For acclimation of the Hsf concentration in the nucleus, import must be balanced by export, and a nuclear export signal (NES) has also been identified in many plant Hsfs. Depending on the rates of import and export into and out of the nucleus, the intracellular distribution of the free Hsfs changes dynamically between the nucleus and the cytoplasm.
Activator elements (AHA motifs)—consisting of aromatic, large hydrophobic and acidic amino acids—are typical of the members of the HsfA class, except for the HsfA8 types. The activator elements can interact with the transcription apparatus and thereby activate it. Similar activator motifs are known from other transcription factors (Baniwal et al. 2004). Hsfs of the B class contain a characteristic tetrapeptide (LFGV, Leu-Phe-Gly-Val) in their C-terminal domains which, by comparison with other transcription factors, is interpreted as a repressor-binding motif. However, the corresponding transcription repressor is not yet known.
Specific roles of the plant heat stress transcription factors: The high diversity and multitude of the principally similar plant Hsfs suggest specific functional roles of these proteins. For instance, in tomato, HsfA1a is a master regulator of the heat stress response and recovery, interacting with Hsfs A2 and B1 in a functional triad upon extended heat stress (Fig. 4.31c). In this triad, HsfA2 functions as an enhancer of heat stress–induced thermotolerance. HsfB1 acts as a synergistic co-activator of HsfA1, whereas in the presence of a co-repressor, class B Hsfs can attenuate the activation of the HS genes. HsfA3 is involved in drought stress signalling, and HsfA9 controls HSP gene expression during seed development (Scharf et al. 2012).
4.3.3.3 Cell Biological Aspects of the Heat Stress Response

Heat stress granula (g) in a tomato cell culture after heat shock (20 → 40 °C within 2 h). (m) mitochondria (Bucka 1999)
4.4 Temperature Sensing

Temperature ranges of plant responses to the ambient temperature; for example, the “bud break” responds to the ambient temperature in a range between 12 °C and 28 °C. “Temperature compensation” refers to the circadian clock. (Modified from Penfield (2008))
Some responses to temperature changes are rapid (e.g. many responses to stressful temperatures), while others depend on the monitoring of temperatures over weeks and months (e.g. vernalisation; Chap. 2). Some processes are controlled by the rate of temperature change, others by the absolute temperature. An example of the latter is the transcriptional activation of CBF genes (Sect. 4.2.5). It is not the cooling that is the trigger but a certain absolute temperature. Another differentiation of temperature perception is that between the sensing of extreme (= potentially damaging) temperatures (frost, cold and heat) and the sensing of non-stressful changes of the ambient temperature within the range of active development (Fig. 4.35).

Involvement of phytochrome in ambient temperature sensing. The kinetics of the P fr inactivation (reversion to P r) in the dark depends on the temperature a and temperature sensing b by phytochrome- and ELF3-dependent regulation of the transcription factor PIF4, which in turn activates the homeobox gene ATHB2 as a master regulator of (hypocotyl) growth of Arabidopsis thaliana (Jung et al. 2016)
4.4.1 Sensing of Extreme Temperatures

Cold and heat shock–induced momentary changes in the cytosolic free Ca2+ concentration in Arabidopsis thaliana. a Repeated cold shocks applied to non-acclimated control plants. b Comparison of Ca2+ signals following a cold shock (21 → 0 °C) in non-acclimated and acclimated (3 days with a cooling period: 3 h at 4 °C) 7-day-old A. thaliana seedlings. In acclimated seedlings the transitory increase in cytosolic [Ca2+] lasts longer (redrawn after Knight et al. (1996)). c Ca2+ signal after a heat shock (21 → 47 °C for 35 min) (Modified from Gong et al. (1998)). Note the different time scales, indicating different sensing mechanisms for cold and heat. Changes in [Ca2+] can be visualised by means of the Ca2+-sensitive luminescent protein aequorin. To that end, transgenic experimental plants stably expressing apoaequorin were used. (Knight et al. 1996)
Evidence exists for distinct Ca signatures (Chap. 2)—that is, specific kinetics of the transient [Ca2+] increases that carry information and enable specificity of the downstream events (Plieth et al. 1999). In mammalian systems, different classes of Ca2+-permeable channels have been identified as essential sensors for cold or heat, respectively. However, no Ca2+ channels orthologous to the ones identified outside the plant kingdom are present in plants, according to genome analyses.
4.4.2 Sensing of Ambient Temperature Changes

H2A.Z and PIF4 as critical components of ambient temperature sensing in Arabidopsis thaliana. Upon an increase in ambient temperature, H2A.Z is evicted from nucleosomes, making DNA more accessible for binding by regulatory factors such as the key regulator PIF4 and activation of genes such as FT. The FT protein (= florigen) triggers a more rapid transition to flowering. (Modified from Kumar et al. (2012))
4.5 Summary
-
Active life requires water in the liquid state, but not the entire temperature range where this applies is equally convenient for organisms. Organisms such as plants, which (apart from a few exceptions) cannot produce heat themselves and thus equilibrate with the ambient temperature, are characterised as either psychrophilic when adapted to low temperatures, as thermophilic when thriving at temperatures above 40 °C and as extremophilic when living in and around hot springs. However, the vast majority of plants are mesophilic, with temperature optima between 20 °C and 30 °C.
-
As so-called poikilothermic organisms, plants can thrive at temperatures above and below the optimal temperature range, experiencing increasing stress with escalating deviation from the optimum. The absolute limits of life—set by cold and heat death, respectively—are generally species-specific. Nevertheless, the habitats colonised by a species can select individuals that are genetically better adapted, yielding ecotypes with different temperature requirements.
-
The vast majority of tropical and subtropical plants are not adapted to cold. Exceptions are tropical alpine plants, which are cold tolerant all year round.
-
Perennial plants bound to habitats that are subject to temperature-induced seasons such as winter and summer can acclimatise. As a result the so-called cardinal points of life (temperature optimum, cold or heat death as points of no return) can shift considerably (by 10–20 °C) to lower temperatures or, less pronounced (<10 °C), to higher temperatures. Seasonal acclimation (hardening and de-hardening) is triggered by environmental cues (e.g. the length of the photoperiod) or by mild forms of the respective stress.
-
Hardening and de-hardening are syndromes encompassing a variety of changes at the biochemical and ultrastructural levels. A principle that applies likewise to cold or heat acclimation is the adjustment of the viscosity (and functionality) of the biomembranes to the altered temperature ranges by an increase (cold hardening) or decrease (acquisition of thermotolerance) in the proportion of desaturated fatty acids in the membrane lipids. This is associated with a change in the expression of the genes encoding fatty acid desaturases.
-
As poikilothermic organisms, plants do not have physicochemical or biochemical thermometers. Nevertheless, they are able to quantify deviations from the optimal temperature range. This kind of temperature sensing is coupled either to the incidence of damage, such as protein inactivation by heat, or simply attainment of a particular temperature range where selected genes are expressed, such as CBF genes in cold hardening. The biochemical mechanisms by which plants can sense temperature are still unknown. However, it appears more likely that there are several thermosensors for particular processes in a plant’s life and acclimation to its environment. One of these is temperature-dependent phytochrome B reversion from the physiologically active Phyfr to the inactive Phyr.
-
An early response to temperature stress is the transient increase of the cytosolic concentration of free Ca2+—that is, its immediate release from cellular pools and fast sequestering into presumably other organelles such as the vacuole. However, such Ca2+ spikes are signals, not thermometers.
-
Apart from the adjustment of the membrane viscosity, the processes of cold hardening/de-hardening and acquisition or loss of thermotolerance differ fundamentally. The impacts of cold are associated not only with a slowdown of (bio)chemical reactions and rigidification of non-acclimatised biomembranes (cold damage by “chilling” at still positive temperatures) but also with a change of liquid water to (hydrophobic) ice at sub-zero temperatures. Intracellular ice formation results in the disintegration of the cellular membranes because of the loss of the hydrophobic interaction of the amphiphilic lipids with liquid water.
-
Therefore, frost hardening triggers either the capability of the cells for extracellular ice nucleation, most probably provoked by so-called antifreeze proteins in the apoplast which, in contrast to their name, control ice formation. A physicochemical equilibrium across the cell membrane between the water potential of ice and that of the cellular solutions results in temperature-dependent progressive cell dehydration by apoplastic crystallisation of cellular water. Freeze dehydration to a residual volume of less than 20% of liquid cellular water is not uncommon.
-
An alternative strategy is an increase in the cell’s capability for supercooling—that is, the avoidance of ice formation by colligatively effective cryoprotectants. These are hydrophilic low molecular weight solutes such as carbohydrates, amino acids and quaternary ammonium compounds which, even at higher concentrations, do not lose their cellular compatibility. Increased production and accumulation of cryoprotectants require a rearrangement of the preferential biochemical routes of the de-hardened state from energy metabolism for growth towards synthesis of less readily metabolisable compounds. Extreme supercooling to the temperature of homogeneous ice nucleation (−40 °C) is known from the xylem tissue of some woody species. However, the mechanisms enabling this physically unlikely phenomenon are not known.
-
The formation of amphiphilic, membrane-protecting proteins (dehydrins), which accumulate also under drought and salt stress, is a common component of the frost-hardening process. Cryoprotectants are produced in plant tissues, which are capable of extracellular ice formation as well. A more negative osmotic potential upon extracellular ice deposition diminishes the degree of freeze dehydration.
-
Cold hardening is accompanied by changes in the chloroplast ultrastructure and a reduction in the photosynthetic efficiency. A substantial decrease in the chlorophyll content indicates the downsizing and even complete degradation of parts of the photosystems. This prevents over-excitation of the photosynthetic light reactions with respect to the utilisation of the end products, reduced nicotinamide adenine dinucleotide phosphate (NADPH) and adenosine triphosphate (ATP). Overstretching of the photosystem’s capacity of de-energisation leads to the formation of reactive oxygen species, causing further damage. Upon de-hardening in spring, the photosystems and antenna complexes are restituted.
-
Not all parts of a plant can harden (and de-harden) to the same extent and at the same time. Roots, old leaves, and growing leaves and twigs are the most temperature-sensitive organs. In the absence of homeostatic temperature regulation, most of the mechanisms involved are operating at the cellular level and protect the tissues directly exposed to an unfavourable temperature.
-
Stress by freezing, drought and high salinity poses osmotic challenges to plants. Accordingly, there is considerable overlap in the molecular responses of cells to these different stress conditions. However, there are also highly complex responses specific to cold acclimation. Many proteins increase in abundance upon exposure to low temperature. They are known as cold-responsive proteins (COR proteins). A large number of COR genes are activated under the control of the CBF transcription factors summarised under the term “CBF regulon”. Binding of CBFs rapidly activates transcription of COR genes. Constitutive strong transcription of CBF genes results in a permanent cold-acclimated state without an exposure to cold. CBF overexpression leads, on the other hand, to a strong reduction in the growth rate as an integral part of stress acclimation responses.
-
Cold acclimation involves rapid up-regulation of the CBF genes. Their expression is controlled by transcription factors that are themselves predominantly controlled at the posttranslational level. A specific transcription factor for cold acclimation is ICE1 which, by binding to cis elements in the CBF promoters, triggers the transcription of CBF genes. Events upstream from ICE1 are less well defined. What is clearly established is a rapid increase in cytosolic Ca2+ levels upon transfer of plants to cold. Another regulatory mechanism leading to enhanced ICE1 activity involves a cold-responsive but Ca2+-independent MAP kinase phosphorylation cascade.
-
Elevated temperatures above the optimal range not only accelerate but also redirect metabolic processes, leading to changes in metabolite pools, thereby affecting growth and many other developmental processes. Apart from such biochemical responses and the aforementioned membrane viscosity problems, denaturation of proteins is the major challenge of heat stress which, for mesophilic organisms, starts at around 35 °C. In many habitats, even in temperate climate zones, temperatures of 40 °C and more are frequently encountered—in particular, during direct sunshine. Even if these events are transitory, they trigger strong heat shock responses, targeted at rescuing misfolded or degrading aggregated proteins in the 26S proteasome. The short-term heat shock must be differentiated from longer-lasting heat stress with a greater potential for heat damage. Both heat shock and heat stress can lead to the so-called acquired thermotolerance: the ability to withstand higher temperatures owing to acclimation.
-
At the cellular level, the major response to a heat shock is the rapid deceleration of housekeeping metabolism, apparent in the down-regulation of housekeeping genes, and the subsequent revival of this metabolism after a return to the normal temperature. During long-term heat stress the housekeeping metabolism has to maintain its function at the elevated temperature. Both sets of reactions, however, depend on the strongly enhanced expression of genes encoding transcription factors (heat shock factors) and heat shock proteins, many of which serve essential cellular functions also in the optimal temperature range.
-
There are several ways in which plants can avoid or at least mitigate heat stress—for example, by timing of sensitive processes and phases such as germination, by dissection of large leaf laminas (e.g. in banana), by covering the leaf surface with a felt of trichomes or by transpiration cooling. Since plants, as sessile organisms, cannot avoid heat stress altogether, all are capable of mounting a response to heat stress.
-
The most important mechanism underlying acquired thermotolerance is the massive production of heat shock proteins (HSPs). However, not only does the expression level of HSP genes change but also up to 2% of all genes are affected in their activity. For many of them the contribution to thermotolerance is functionally not understood. What is evident is an up-regulation of anti-oxidative defences to counteract the increased production of reactive oxygen species under heat stress. Also, the concentrations of compatible solutes increase, which can protect proteins from unfolding and thus shift the onset of the heat stress response to higher temperatures.
-
The classical heat shock reaction requires stress-induced accumulation of misfolded proteins activating heat shock transcription factors (Hsfs), which then bind to specific elements (heat shock elements (HSEs)) in the promoters of genes encoding heat shock proteins (HSPs). HSPs—named after their enhanced production upon heat treatment—are essential components of both prokaryotic and eukaryotic cells for life and stress survival as well. HSPs act as chaperones required for correct folding of nascent proteins upon release from the ribosome or after stress-induced misfolding, unfolding or aggregation of unfolded proteins. They do not exhibit enzymatic activity and are classified by their molecular weights. The two types (prokaryotic and eukaryotic) differ in the details of their modes of action in the so-called HSP cycle. The misfolded protein is bound via an exposed hydrophobic stretch to the interior (also hydrophobic) domain of the HSP which, by consumption of ATP, is converted into a hydrophilic micro-environment; this in turn forces the misfolded protein back into its natural conformation with a hydrophilic surface. After release of the repaired protein, the HSP is ready for a new round either with the same protein (if the repair was not yet complete) or with another misfolded protein. Several co-chaperones are required for the function of the HSP cycle.
-
Chaperones are, in addition, involved in the removal of irreversibly denatured proteins. The function of HSPs in the removal of such proteins is the targeting of the poly-ubiquitinated candidates to the 26S proteasome.
-
The normal heat shock response starts with the transcriptional activation of HSP genes by Hsfs. Hsfs have a modular structure, which is conserved in the entire eukaryotic kingdom. Plant genomes are particularly rich in Hsfs: in Arabidopsis thaliana 21 Hsf genes, in soybean even 52 Hsf genes are known. Hsfs mediate the acute heat shock response, as well as the acquisition of thermotolerance. Under normal conditions, Hsfs are inactive because of their association with heat shock proteins, which prevent their oligomerisation. Heat denaturing of proteins results in dissociation of the chaperone–Hsf complexes because of the association of the HSPs with the mis- or unfolded proteins. The liberated Hsfs oligomerise and bind to the heat stress elements (HSEs) in the promoters of heat stress genes, which usually harbour more than one HSE. The transcriptions factor HsfA2 has turned out to be one of the key elements in the plant stress response. It is involved in protection against heat, reactive oxygen species, salt stress and even anoxia, and accumulates to high levels upon long-term heat stress. The interaction between heat shock factors and heat shock proteins exerts an effective control over the up-regulation of HSP synthesis. The presence of more denatured proteins than under non-stressed conditions triggers the interaction of Hsfs with HSP gene promoters, and a decline in the number of denatured proteins gradually tunes out the extra HSP synthesis.
-
In the heat shock response, sensing of high temperature occurs via the abundance of inactivated, misfolded or even aggregated proteins. A second mode of sensing of protein denaturation has been described for the endoplasmic reticulum (ER), where one of the sensors is an ER membrane–associated basic leucine zipper protein, bZIP28, as a transcription factor for chaperones. It is arrested in the ER by association with the major ER chaperone, the “binding immunoglobulin protein” (BIP). Upon accumulation of misfolded proteins in the ER, BIP is competed away from bZIP28 and associates with the accumulating misfolded proteins. After several modifications, bZIP28 enters the nucleus as a transcription factor binding to the heat shock elements in the promoters of heat shock genes.
-
Organisms can acquire thermotolerance by maintaining a high level of HSPs while the normal “housekeeping metabolism” continues. During an acute heat shock, in contrast, the cell redirects its activity to almost exclusive synthesis of HSPs. Processing of the pre-messenger RNAs (pre-mRNAs) of the housekeeping genes is stopped with the preferential translation of the heat stress gene mRNAs. To avoid degradation of unprocessed pre-mRNAs and thus allowing immediate resumption of housekeeping gene expression, the pre-mRNAs associated with heat shock proteins are conserved in the nucleus or the surrounding cytosol in the form of heat stress granula.