
Mixed deciduous forest in Central Europe, Hainich National Park. (Photo: courtesy of S. Getzin)
“I fly window seat, incurably. It doesn’t matter that I’ve been on countless flights over the past four decades and stared downward over numberless vistas of forest and wetland, prairie and river. When the flight attendants ask for people to lower the window shade so that you can see the movie better, that’s my shade still up, my face pressed against the glass.
I revel in the details of geography and sweep of biology that can be seen from the air. I trace trails into box canyons and along mountain ridges; search tundra for oriented lakes; look at sediment roiling downcast from a turbulent river mouth; scan bayous for cypress islands; enjoy the dendritic meanderings of channels through a tide flat; discover oxbow lakes along the fringes of a river basin.
Anyone who shares this affliction with me knows, however, that what one sees most of the time along too many common routes is the human footprint: dams and dikes, farmland, shopping malls and roads, hills bulldozed, forests flattened, massive works of civil (and uncivil) engineering.”
John Peterson Myers (Myers 1997, page xvii)
20.1 Introduction
Biological diversity—or biodiversity —is one of the most striking elements of planet Earth and has always fascinated people. By provisioning food, shelter and all materials needed for survival, biodiversity has been the very foundation of human life for thousands of years. Perhaps not surprisingly, early human cultures obviously adored the many creatures living with them, and they left remarkable legacies of their view of life in the oldest human cave paintings, dating back to the Upper Palaeolithic, approx. 17,000 years BC. The many naturalists and artists travelling around the world in the seventeenth and eighteenth centuries also beautifully documented the astonishing diversity of life as they entered new territories. Even today, biodiversity is an inexhaustible source of inspiration and creativity in arts, and many people make excursions to watch birds and plants or to simply enjoy being outside in nature. The affinity towards and admiration of the rich diversity of life forms and ecosystems around us are obviously fundamental features of human life, which has been recognised as the biophilia hypothesis by Erich Fromm (1964) and Edward O. Wilson (1984).

Conceptual framework of this chapter. The biotic community can be characterised by different biodiversity components (Sect. 20.2). Biodiversity is controlled by the environment (Sect. 20.3) but also affects ecosystem functioning (Sect. 20.4). Interactions within biotic communities are described in Chap. 19, while influences and feedbacks between ecosystem services, human activities, global changes and ecosystems are dealt with in Chap. 23. Solid lines represent direct influences and the dotted line shows a feedback loop. Modified from Hooper et al. (2005), reproduced with permission from John Wiley & Sons
Beyond science, biodiversity attracted the interest of the general public following the Earth Summit in Rio de Janeiro in 1992, as a result of which the conservation of biodiversity, the sustainable use of its components and the fair and equitable sharing of the benefits arising from the utilisation of genetic resources became regulated (United Nations Convention on Biological Diversity, CBD ). People from all walks of life, including politicians, became aware of the global loss of species, a loss caused by a situation in which human influences grew to become significantly greater than natural rates of extinction. Scientists recognised that knowledge of biodiversity was rather modest and feared that numerous organisms would become extinct without ever having been scientifically recorded. Since that time, research on the functional consequences of biodiversity has gained strong momentum. Mere numbers of species were not regarded sufficient to understand communities in their habitats; it was recognised that other aspects of biodiversity, such as the functional characteristics of species, also play an important role in how ecosystems work. Plants and animals were to be regarded not only as resources but as decisive factors controlling processes in ecosystems.
Currently, ecosystems are being increasingly disturbed by different drivers of global change , including altered biogeochemical cycles and eutrophication, changing land use and its intensity, changing climate and biotic changes (Chap. 23). In consequence, habitats and species are being lost and gained, while new conditions are created by human interference; for instance, human management is responsible for the previously unknown diversity in traditional agricultural landscapes of central Europe. Therefore, it is very urgent to clarify important questions on the development, loss and function of biodiversity.
This chapter presents the various facets and definitions of biodiversity, focusing on compositional, structural and functional aspects, where also the concept of plant traits will be introduced (Sect. 20.2). In a subsequent part, observed patterns of biodiversity in response to environmental factors will be presented (Sect. 20.3). Finally, Sect. 20.4 discusses the effects that biodiversity changes have on the properties and functions of ecosystems. The effects of management and human activities are dealt with in Chaps. 17 and 23.
20.2 Various Facets of Biodiversity
Mostly, the term biodiversity is used as a synonym for species richness, both in the media, but also still often in science. While early biodiversity research focused mainly on the number of species in an area, biodiversity is now seen as a broad concept, and it has been defined in numerous ways (Heywood and Watson 1995). In essence, it encompasses the variability of biological entities across all levels of biological hierarchies, that is, from the level of genes to ecosystems. Edward O. Wilson has simply coined it the “variety of life” (Wilson 1992).
Hierarchical organisation of biodiversity
Genetic diversity |
Organismic diversity |
Ecological diversity |
---|---|---|
Kingdoms |
Biomes |
|
Phyla |
Bioregions |
|
Families |
Landscapes |
|
Genera |
Ecosystems |
|
Species |
Habitats |
|
Subspecies |
Niches |
|
Populations |
Populations |
Populations |
Individuals |
Individuals |
|
Chromosomes |
||
Genes |
||
Nucleotides |
Indicators and measures of biodiversity
Indicators |
|||
---|---|---|---|
Composition |
Structure |
Function |
|
Regional- landscape |
Identity, distribution, richness, and proportions of patch (habitat) types and multipatch landscape types; collective patterns of species distributions (richness, endemism) |
Heterogeneity; connectivity; spatial linkage; patchiness; porosity; contrast; grain size; fragmentation; configuration; juxtaposition; patch size frequency distribution; perimeter-area ratio; pattern of habitat layer distribution |
Disturbance processes (areal extent, frequency or return interval, rotation period, predictability, intensity, severity, seasonality); biogeochemical fluxes, nutrient cycling rates; energy flow rates; patch persistence and turnover rates; rates of erosion and geomorphic and hydrologic processes; human land-use trends |
Community- ecosystem |
Identity, relative abundance, frequency, richness, evenness and diversity of species and guilds; proportions of endemic, exotic, threatened and endangered species; dominance-diversity curves; life-form proportions; similarity coefficients; C4:C3 plant species ratios |
Substrate and soil variables; slope and aspect; vegetation biomass and physiognomy; foliage density and layering; horizontal patchiness; canopy packing, openness and gap proportions; abundance, density and distribution of key physical features (e.g. cliffs, outcrops, sinks) and structural elements (snags, down logs); water and resource (e.g. mast) availability; snow cover |
Biomass and resource productivity; herbivory, parasitism, and predation rates; colonisation and local extinction rates; patch dynamics (fine-scale disturbance processes); biogeochemical fluxes, nutrient cycling rates; human intrusion rates and intensities |
Population- species |
Absolute or relative abundance; frequency; importance or cover value; biomass; density |
Dispersion (microdistribution); range (macrodistribution); population structure (sex ratio, age ratio); habitat variables (see community-ecosystem structure, above); within-individual morphological variability |
Demographic processes (fertility, recruitment rate, survivorship, mortality); metapopulation dynamics; population genetics (see below); population fluctuations; functional traits; physiology; life history; phenology; growth rate (of individuals); acclimation; adaptation |
Genetic |
Allelic diversity; presence of particular rare alleles, deleterious recessives or karyotypic variants |
Census and effective population size; heterozygosity; chromosomal or phenotypic polymorphism; generation overlap; heritability |
Inbreeding depression; outbreeding rate; rate of genetic drift; gene flow; mutation rate; selection intensity |
Clearly, these broad descriptions of the term are not very helpful for more specific questions such as whether an ecosystem is functioning in different ways if biodiversity is lost. Thus, biodiversity must be defined more specifically for any given study, for example, by referring to a specific element listed in Table 20.1; these elements are hierarchically nested within each group.

Three spheres of biodiversity. Biodiversity can be categorised into compositional, structural and functional spheres that are interconnected. Each sphere encompasses multiple levels of organisation (Noss 1990). Reproduced with permission from John Wiley & Sons
20.2.1 Compositional Diversity

Differences in plant species richness. Plant communities differ largely in species richness depending on environmental conditions, evolutionary history or human interference. a Species-rich natural, moist tropical forest with more than 8000 mm precipitation per year in the Bach Ma National Park in the central highlands of Vietnam, at 1000—1400 m a.s.l. b Coniferous forest in the Mediterranean region dominated by only one tree species (Pinus halepensis). In both cases, there has been little human interference in the forests. (Photos: E. Beck, K. Müller-Hohenstein)
While the number of distinct species per area or of an entire ecosystem (= species richness or species density) is by far the most frequently used metric, it should be kept in mind that biodiversity not only comprises the number of species. In fact, there is no single measure of biodiversity, and it is impossible to define the biodiversity of an ecosystem (Gaston and Spicer 1998). However, species richness has turned into a kind of “currency” in biodiversity research, because it is relatively easy to measure and because species differ in their genetic composition and functional traits, in their growth forms and stature, in their habitat requirements and biocoenotic interactions. Therefore, species diversity can also be seen as a “surrogate” for certain facets of genetic, organismal, structural, functional and ecological diversity. Species diversity and genetic diversity, for example, are sometimes well correlated, presumably because the processes determining genetic diversity should also determine species diversity. However, this covariation is mainly found in small-scale, isolated ecosystems such as oceanic islands or forest patches. In contrast, genetic diversity might be more related to demographic history in more widespread, well-connected populations where these two facets of biodiversity are less correlated (Taberlet et al. 2012).
The strong focus on species diversity calls for a proper designation of the species concept, which is not as easy as one might expect (Box 20.2). In what follows, we will also focus on the species level to illustrate some global patterns of compositional diversity.
Box 20.1: Quantification of Biodiversity
How diverse is an ecosystem, and does this diversity change over time? Do distinct landscapes differ in their biodiversity? Which aspect of biodiversity responds first to changes in environmental conditions or management? To answer such typical questions in ecology, biodiversity must be quantified. However, the concept of biodiversity with its numerous facets is difficult to handle without proper definition and measures.
In essence, any measure of biodiversity should encompass the richness of different entities, and the degree of difference or dissimilarity between those entities, for example, species in a sample or in a community. One way to represent the differences between such entities is to account for the relative abundance or evenness of those entities. Other ways to differentiate between entities include aspects of genetics, morphology, biochemistry, biogeography or the functional role within ecosystems. Therefore, it has to be noted that “there is no single all-embracing measure of biodiversity—nor will there ever be one!” (Gaston and Spicer 2004).
The measure of species richness is simply the total number of species within a sample or community. The number of individuals of each species is irrelevant for this measure. If the number of species is related to an area (e.g. per square metre), the term species density is also used.
To distinguish diversity at different spatial scales, the concept introduced by Whittaker (1960) is often used, which distinguishes the following categories:
Point diversity: number of species in a small or micro-habitat sample, taken within a community regarded as homogeneous;
α-diversity : within-habitat diversity, that is, the number of species within a community and per area;
β-diversity : between-habitat diversity differentiation, that is, a dimensionless comparative number of species in different units of vegetation or habitats;
γ-diversity : landscape diversity, that is, the number of species in larger units, such as an island or landscapes that include more than one community.
Later, he added two levels at higher spatial scales, which, however, are rarely used:
δ-diversity: geographic diversity differentiation, that is, dimensionless comparative number of species applied to changes over large scales (e.g. along climatic gradients or between geographic areas); it is the functional equivalent of beta diversity at the higher organisational level of the landscape;
ε-diversity: regional diversity, that is, the total number of species in a broad geographic area, including different landscapes or groups of areas of gamma diversity.
α-, γ- and ε-diversity are thus measured in particular areas, the first in those that are occupied by a community, the latter in larger spatial units, such as ecosystems, landscapes or regions. β- and δ-diversity, in contrast, must be calculated and describe changes in the number of species between habitats or landscapes or along ecological gradients. β-diversity may also be used for comparisons of number of species in the same habitat over time and is then a measure of species turnover .
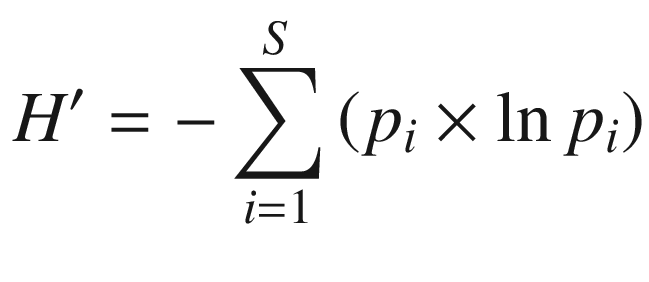
where
H′ Shannon’s diversity index,
S number of species present in sample,
p i relative frequency per area (abundance) of ith species, measured from 0–1,
n i number of individuals of species i,
N total number of individuals.
In this index, the richness and proportional abundance of species are included because both aspects determine the heterogeneity—and therefore the diversity—of a community. H′ rises with an increasing number of species or increasing uniformity of distribution of relative abundance (e.g. cover or biomass) of individual species. In habitats with single species, the value is zero. If all species have the same relative abundance, H′ is at its maximum. Shannon’s index is independent of the functional characteristics of the individual species: all are treated equally.
The same index can also be used to calculate the genetic diversity within a population, with p i being the relative frequency of the ith allele.

where H′ max is the maximum value of
H′ and equal to

To obtain an increasing value with increasing diversity, this index is most often presented as Simpson’s index of diversity 1 − D (ranging from 0 to 1) or as Simpson’s reciprocal index 1/D.
To describe the similarity or dissimilarity in species composition between different floristic regions, plant communities or sample quadrats, several indices have been developed, for example:

a number of species common to both samples,
b number of species unique to the first sample,
c number of species unique to the second sample.

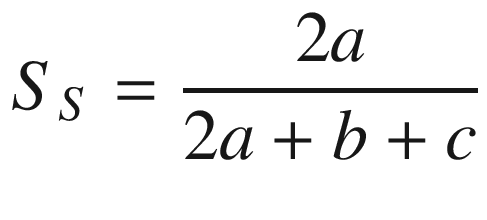
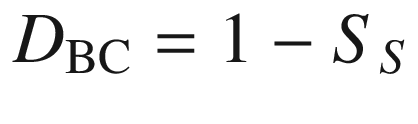
This index is bound between 1 and 0, with 1 representing a situation where the two samples do not share any species, and 0 meaning the two samples have identical species compositions.
Other indices to compare species compositions have been developed, for example, by including information on abundances, by generalising to more than two samples or by using other distance measures such as Euclidean distances. More details can be found in textbooks dealing with analyses of ecological data (e.g. Legendre and Legendre 2012).
Box 20.2: The Species Concept
A species is the central taxonomic rank of biological systematics, and species are one of the fundamental units in biology, similar to genes, cells and organisms. Species names are binomial, meaning they include the genus (e.g. Abies) and the species rank (e.g. alba), when naming silver fir, for instance. In botany, species can be further subdivided into subspecies, varieties and forms (so-called infraspecific taxa). The way to name species is defined in the “International Code of Nomenclature for algae, fungi, and plants (Melbourne Code)”, adopted by the Eighteenth International Botanical Congress Melbourne, Australia, July 2011.
Despite this clear taxonomic classification, it is not always easy to determine what a species actually is. A central question related to species concepts is thus the determination of clear boundaries between species, which must be based on well-defined criteria: What degree of morphological differences is enough to separate morphospecies? How large must the genetic difference be to separate two species? Evolutionary processes (speciation) are dynamic and ongoing, and so boundaries between species are constantly shifting. In principle, to separate a group of individuals into two species, the variability of genotypes or phenotypes between species should be larger than between and within subpopulations within a species. However, answers to such questions have changed over time, and the nomenclature therefore also sometimes changes with the emergence of new knowledge. For example, major shifts in taxonomic units, also at the levels of families or orders, took place following the adoption of molecular techniques. Thus, species names are primarily a hypothesis, which can change if new data become available.
While early definitions of species were mostly based on similarities in morphological or other observable traits (morphological or phenetic species concept), the biological species concept defines a species as “groups of actually or potentially interbreeding natural populations, which are reproductively isolated from other such groups” (Mayr 1942). This concept works well in most cases, especially for sexually reproducing organisms. However, for plants, the biological species concept is somewhat problematic because of hybridisation between species, rarely also between genera (e.g. Triticale), and in the case of asexually reproducing organisms, for example, in apomictic plants (e.g. Rubus). More than 20 different species concepts have been proposed, including an ecological species concept (emphasising the occupation of ecological niches) and evolutionary or phylogenetic concepts. In particular, the use of modern molecular techniques opened the door to distinguishing taxonomic units based on genetic distances. All these definitions are based on distinct biological properties, which are acquired by lineages during the course of divergence (e.g. phenetically distinguishable, reproductively isolated, monophyletic) (de Queiroz 2007). Some concepts are partially incompatible with each other (often referred to as the “species problem”), and the adoption of different concepts allows for the occurrence of different species boundaries and, thus, different numbers of species. Nevertheless, the biological species concept is still the most often used species concept.
A proposed solution to the species problem suggests that the only necessary property of a species is the existence of a separately evolving metapopulation lineage (i.e. an ancestral–descendant sequence of sets of subpopulations connected through the exchange of genes; such groups of connected subpopulations are termed metapopulations). This property is inherent to all contemporary species concepts (de Queiroz 2005). It is a further development of Darwin’s revolutionary idea to see species as branches in the line of descent, that is, the evolutionary basis for the concept of species. All other proposed properties to qualify being a species, such as being phenetically distinguishable, monophyletic (i.e. a group of species with an ancestral species and all its descendants), reproductively isolated or ecologically divergent, are considered as secondary, contingent properties of a species. These properties may (or may not) be acquired during the course of species existence and can be seen as different operational criteria to assess lineage separation and, hence, as evidence for the existence of a species. This general and unified species concept also works for asexually reproducing species if they form metapopulations as the result of some processes other than the exchange of genetic material or interbreeding, for example, by natural selection.
Estimates of the total number of all species on Earth vary greatly. Because many regions are not well sampled, we do not have a direct quantification of the global number of species. Instead, several indirect estimates have been put forth, all of which rely on particular assumptions and, therefore, have limitations. Current estimates range between three and ten million species, but the actual figure may be higher by a factor of ten. Approximately 225,000 vascular plant species have been taxonomically described, but the total number could be around 315,000 (Mora et al. 2011).

Management and biodiversity. High species diversity is often found in traditionally cultivated landscapes, irrespective of climatic conditions. a Large number of cultivated rice varieties in a rural Indonesian market (Palu, Sulawesi). b A home garden in southern Chile (Puerto Montt). In both cultivated areas agrochemicals and modern agronomic techniques have little impact. (Photos: K. Müller-Hohenstein)
Staying at the species level, compositional diversity encompasses not only species numbers but also the frequency and abundance of different species, that is, species composition . Obviously, two plant communities may have the same species number but harbour very different species in different abundances. Such differences in species composition can be either documented in phytosociological tables (full information retained), or by visualising species abundance distributions , for example, as rank-dominance curves (moderate retention of information), or by calculating indices of similarity (easy to understand but high loss of information, for example, Jaccard or Sørensen coefficients, Box 20.1). Multivariate statistical tools can also help to visualise differences in species composition (e.g. showing the range of species composition along environmental gradients). Species composition has big consequences for the diversity of other organismic groups, such as pollinators, herbivores or pathogens, since many higher trophic groups are to varying degrees specialised for certain plant species (Sect. 19.4). In addition, species composition also has large effects on ecological processes, such as nutrient cycling, because species differ in their morphological, physiological and chemical properties or traits (Sect. 20.2.3).
20.2.2 Structural Diversity
Looking at a plant community it becomes obvious that the species present may build up different structures, that is, different physical patterns. Vegetation structure is not only an important driver of the diversity of an ecosystem; it is also the key aspect that couples plants to the atmosphere, especially for gas exchange, trace gas fluxes, atmospheric deposition and rainfall interception (Sects. 9.1, 16.1–16.3).
Categories of plants by life form
Humboldt (1806) |
Drude (1887) |
Raunkiaer (1908) |
Schmithüsen (1968) |
|
---|---|---|---|---|
Palms |
Woody plants with leaves |
Phanerophytes |
Herbaceous plants |
Trees with crowns |
Banana form |
Trees |
Chamaephytes |
Annuals |
Trees with apical bunched leaves |
Mallow form |
Bushes |
Hemicryptophytes |
Biennials |
Giant grasses |
Mimosa form |
Lianas |
Cryptophytes |
Deciduous perennial |
Strangler figs |
Heathers |
Mangroves |
Therophytes |
Evergreen perennial |
Lianas |
Cactus form |
Parasites on woody plants |
Shrubs |
||
Orchids |
Leafless woody plants |
Subdivided after |
Dwarf trees |
|
Casuarinas |
Stem succulents |
Leaf size |
Woody plants |
Stem succulents |
Pines |
Leafless bushes |
Leaf duration |
Deciduous |
Herbaceous plants |
Arum form |
Small bushes |
Vegetative reproduction |
Evergreen |
Epiphytes |
Lianas |
Perennial herbs |
Dwarf shrubs |
||
Aloes |
Rosette plants |
Small bushes |
||
Grass form |
Leaf succulents |
Dwarf succulents |
||
Ferns |
Epiphytes |
Chamaephytic perennial herbs |
||
Lilies |
Hapaxanthic plants |
Hemicryptophytic woody plants |
||
Willow form |
Land plants |
Hemicryptophytic perennial herbs |
||
Myrtle form |
Water plants |
Winter annuals |
||
Melastoma form |
Lichens |
Geophytic perennial herbs |
||
Laurel form |
Saprophytes/parasites |
Therophytic herbs |
||
Floating leaf plants |
||||
Submerged herbs |

Structural diversity and species richness. A tropical rain forest in Indonesia a, rich in species and in structures, has a structural diversity that is similar to that of an extra-tropical rain forest b, poor in species but rich in structure. However, the number of higher plant species differs ten-fold between both forest types. Cultivated cereal fields are single layered and as such poorer in structure than forests. Nevertheless, there are large differences in species richness among differently cultivated fields: a species-rich but structure-poor fallow c and a species- and structure-poor cereal field d, both located in Mediterranean areas of Morocco. (Photos: K. Müller-Hohenstein)

Structural diversity in plant communities often begets biodiversity of other organisms. Bird diversity in temperate, deciduous forests of north-eastern North America is positively correlated with the structural diversity of the tree canopy. Foliage height diversity is a measure of habitat structural complexity and has been calculated as Shannon’s diversity index with the proportion of the total foliage in several horizontal layers. Bird species diversity is Shannon’s diversity index of breeding bird species territories recorded per 5 acres (approx. 2 ha). Two data points from a tropical savanna and from a pure spruce forest are also included. (MacArthur and MacArthur 1961). Reproduced with permission from John Wiley & Sons
The structure of a plant community is also very relevant for the coupling between the biosphere and the atmosphere: the roughness of a canopy largely determines the exchange of water vapour and CO2 (Sects. 16.1 and 16.2) and strongly influences the microclimate (Sect. 9.2). For example, the daytime surface temperature of a forest near the alpine tree line is much lower than that of adjacent alpine grassland because the tall trees are coupled to the lower atmospheric layer, causing a transport of convective heat (Sect. 9.1). In contrast, small grasses and herbs are decoupled from atmospheric conditions, leading to a strong warming of the grassland canopy and top soil during periods of high radiation (Körner 2003).
In landscape ecology, where the importance of scale is in focus, biodiversity and the diversity of abiotic conditions or environmental heterogeneity are summarised as landscape diversity . Abiotic features of landscapes and the spatial heterogeneity and arrangement of structures are very important for biodiversity, and landscapes differ in biodiversity. It can be assumed that landscapes with spatially heterogeneous abiotic conditions may provide a larger variety of potential niches than those with more homogeneous conditions. For example, Schulze et al. (1996) showed that the richness and diversity of trees and shrubs were significantly higher at sites with high geomorphological heterogeneity than at sites that exhibited little change in terrain or soil conditions in deciduous forest ecosystems. At the landscape scale, human-created structures must also be considered, for example, fragments of almost natural stands or linear elements such as hedgerows and windbreaks. At this level it is clear that structural heterogeneity contributes to high diversity and that diversity changes greatly in transitional areas of different ecosystems (ecotones), for species as well as for structures.
20.2.3 Functional Diversity
“In the quest to understand species coexistence and community assembly, and to address the ecological consequences of anthropogenic changes, ecologists have moved from counting species to accounting for species” (Cadotte et al. 2013, p. 1234, italics added). This insight derives from the fact that species largely differ ecologically and play different roles or functions in ecosystems. That is, besides species richness, evenness and composition, the ecological dissimilarity or functional differences among species cover an important aspect of biodiversity. To describe the dissimilarity among biological entities with respect to their functional roles in ecosystems, different measures of functional diversity have been developed. Functional diversity relates to “those components of biodiversity that influence how an ecosystem operates or functions” (Tilman 2001). It has increasingly been used to study the consequences of biodiversity change for ecosystem functioning and the delivery of ecosystem services (for a definition of these terms, Box 20.4) because it includes information on species characteristics—or traits—that are assumed to control ecological processes (Sect. 20.4).
In essence, any measure of functional diversity will summarise the value, range, distribution and relative abundance of traits in a community. In other words, indices of functional diversity capture the amount of variation in a multivariate trait space or hypervolume represented by the species (or other biological entities) within a community. This trait hypervolume thus characterises the phenotypic space occupied by a set of species. Therefore, it is important to get a clear understanding of the meaning of functional traits . Traits are characteristics of plants at the scale of cells, tissues and up to the whole organism that reflect their evolutionary history and that shape their performance. Hence, traits are rather broadly defined and encompass heritable quantitative morphological, anatomical, biochemical, physiological or phenological properties of organisms (Garnier et al. 2015). These properties must be measurable at the individual level. Traits can be continuous (e.g. specific leaf area, seed size) or categorical (e.g. life form, leaf habit). They have a direct or indirect impact on the fitness of an individual plant through their effects on growth, reproduction and survival, which constitute the three components of plant performance. Thus, traits offer insights into questions such as (Reich 2014): How and why does a plant “behave” as it does? Where does a plant grow and where does it not grow? How does a plant interact with other plants or other organisms, such as herbivores? How does it influence the abiotic and biotic environment around it? Analysing such questions from the perspective of plant attributes has therefore been called a trait-based approach to plant ecology, based on the work of Humboldt, Schimper, Larcher and many others.
Many traits are often correlated or covary across species or are similar in their functional consequences. These trait syndromes capture fundamental trade-offs along several important axes of plant strategy and function. Three dimensions of plant ecological strategies are considered fundamental for understanding plant functioning: the acquisition and use of resources, the stature of the plant and the capacity for sexual reproduction. For above-ground parts, these dimensions are represented by the leaf economics , canopy size and seed size spectrum (leaf–height–seed scheme) (Westoby 1998). For example, plants may have “cheaply constructed” leaves, with low leaf mass per area (LMA) (kg m−2) resp. high specific leaf area (SLA) (m2 kg−1), that have a short lifespan but high N and P concentrations and gas exchange rates (Sect. 12.3). Among trees, European aspen (Populus tremula) would be an example. At the other extreme of this range, plants invest more biomass per leaf area (high LMA, low SLA), resulting in leaves with long lifespans, often associated with high concentrations of secondary metabolic compounds but low N and P concentrations and gas exchange rates. Norway spruce (Picea abies) would be an example for this strategy. Plant stature is related to the competitive ability of species: being larger than neighbours confers a competitive advantage in light capture and is therefore an important characteristic of a carbon acquisition strategy (Sect. 12.5). European beech (Fagus sylvatica), for instance, can grow in the shade of neighbouring trees for a long time, but it ultimately outcompetes other species owing to its large maximum height at maturity. The seed-size trade-off involves plants with large individual seed sizes but a low number of seeds or seed output per canopy area; such plants tend to have higher seedling survival under intense competition or low resource availability. An example would be sweet chestnut (Castanea sativa). Such species follow a so-called K strategy , living in densities close to carrying capacity (K) and producing fewer seeds (Sects. 17.3 and 19.3). In contrast, plants with small seeds can produce many more seeds with the same relative investment, enhancing dispersal to sites with low competition or high resource availability (Sect. 18.2). An example of such a species with high growth rates (r) and high seed output (r strategy ) would be silver birch (Betula pendula).
In addition to these three major axes of ecological strategy, other trait dimensions with large variation are also important for plant functioning, including xylem hydraulic and mechanical property trade-offs of stems or wood, which is especially important for tree performance (Westoby and Wright 2006). In trees, the xylem is mainly responsible for the transport of water and nutrients, but also for mechanical stiffness. Therefore, a trade-off between conductive efficiency and mechanical strength can be found. In addition, conductance also trades off with resistance to embolism (i.e. the formation of gas bubbles in vessels, blocking the movement of water) because larger vessels or wider pit pores have a higher risk of embolism (Sect. 10.2).
More recently, the idea of a general whole-plant fast–slow or acquisition–conservatism spectrum of plant economics has been developed. It states that rates of resource acquisition and processing are converging for roots, stems or leaves owing to strong selective pressure along resource axes (Reich 2014). In other words, plant species that are fast in acquiring carbon (leaves), water or nutrients (roots) must also have characteristics enabling fast rates or use at other organs. For example, species that are capable of moving water rapidly also have low tissue density, short tissue lifespans and high rates of resource acquisition and flux at organ and individual scales. The converse is generally found for species with a slow or “conservatism” strategy. The fast–slow spectrum of traits also scales up to the ecosystem level, where the dominance of species with a fast strategy is associated with faster rates of ecosystem processes such as productivity or decomposition of organic matter, and vice versa (Sects. 12.5 and 16.2).
Root traits have been investigated less, but they are of course essential for water and nutrient uptake, anchoring, storage and competitive ability. Interestingly, some below-ground traits seem to covary with above-ground traits in some species, such as rooting depth and maximum height or specific root length (SRL) and SLA. However, for other species, coordination between above- and below-ground traits has not been found, which can be explained by the very different nature of the above- vs. below-ground environment and the different functions of roots, stems and leaves: roots must acquire water and nutrients from the soil solution, stems must provide mechanical strength for height growth and transport, and leaves must capture light and allow gas exchange for photosynthesis.
In addition, the presence of mycorrhizae in most plants also changes many root traits, which makes trait coordination between above- and below-ground traits even less likely (Sect. 7.4).
Association of selected traits with plant responses to environmental changes, plant competitive strength and protection against herbivores and pathogens, and effects on biogeochemical cycles and disturbance regimes
Climate response |
CO2 response |
Soil resource response |
Distur-bance response |
Competi-tive strength |
Plant defence/protection |
Effects on biogeo-chemical cycles |
Effects on distur-bance regime |
|
---|---|---|---|---|---|---|---|---|
Whole-plant traits |
||||||||
Growth form |
● |
● |
● |
● |
● |
● |
● |
● |
Life form |
● |
● |
● |
● |
● |
● |
● |
|
Plant height |
● |
● |
● |
● |
● |
● |
● |
● |
Clonality |
● |
? |
● |
● |
● |
? |
||
Spinescence |
● |
? |
● |
● |
? |
|||
Flammability |
? |
● |
? |
● |
● |
|||
Leaf traits |
||||||||
Specific leaf area |
● |
● |
● |
● |
● |
● |
||
Leaf size |
● |
? |
● |
● |
● |
● |
||
Leaf dry matter content |
● |
? |
● |
● |
● |
● |
||
Leaf N and P concentration |
● |
● |
● |
● |
● |
● |
● |
|
Physical strength of leaves |
● |
? |
● |
● |
● |
● |
||
Leaf lifespan |
● |
● |
● |
● |
● |
● |
● |
● |
Leaf phenology |
● |
● |
● |
● |
● |
|||
Photosynthetic pathway |
● |
● |
● |
|||||
Leaf frost resistance |
● |
● |
● |
|||||
Stem traits |
||||||||
Stem specific density |
● |
? |
? |
● |
● |
● |
● |
|
Twig dry matter content |
● |
? |
? |
? |
● |
● |
● |
|
Twig drying time |
● |
? |
? |
? |
● |
|||
Bark thickness |
● |
● |
● |
? |
||||
Root traits |
||||||||
Specific root length |
● |
? |
● |
● |
● |
? |
||
Diameter of fine root |
● |
? |
● |
|||||
Distribution of rooting depth |
● |
● |
● |
● |
● |
● |
● |
|
95% rooting depth |
● |
? |
● |
● |
● |
|||
Nutrient uptake strategy |
● |
● |
● |
● |
● |
● |
||
Regenerative traits |
||||||||
Dispersal mode |
● |
|||||||
Dispersal shape and size |
● |
|||||||
Seed mass |
● |
● |
● |
● |
||||
Resprouting capacity |
● |
● |
● |
● |
Predicting ecosystem properties by plant traits
Biomass-weighted mean traits |
|||
---|---|---|---|
SLA |
LDMC |
LNC |
|
NPPSA |
0.78** |
−0.71** |
0.87*** |
Litter mass LossSM |
0.78** |
−0.81** |
0.74* |
CSoil |
−0.88*** |
0.84*** |
−0.96*** |
NSoil |
−0.84*** |
0.83*** |
−0.93*** |
Plants are immobile, meaning they cannot physically escape predators and pathogens, search for pollinators or hide from approaching extreme climatic situations. Obviously, plants as sessile organisms must continuously cope with changing environmental conditions—from minute-by-minute, daily, seasonal to decadal fluctuations in temperature and light, changes in nutrient and water availability, or herbivore pressure, pathogen load or loss of mutualistic partners. Plants have therefore evolved a variety of mechanisms that enable them to tolerate and withstand environmental change, and to re-achieve internal homeostasis: plants are highly plastic and resilient to cope with a highly dynamic environment. This phenotypic plasticity , or the capacity of a given genotype to produce different physiological or morphological phenotypes in response to different environmental conditions, is also reflected in plant traits. Traits are usually quantified comparatively across species and are highly context-dependent. This means that traits are not only genetically fixed and heritable properties, but are also plastic. They can change over time (e.g. seasonal or ontogenetic variations) and can depend on environmental conditions, the presence of competitors or herbivores and pathogens. Examples of this phenotypic plasticity of traits are the development of sun and shade leaves within a single crown (Sect. 3.2) or the increase in defensive metabolites after herbivore attack (Sect. 8.3). In fact, plasticity can be considered a trait of its own, given its large variability across species and habitats. Trait plasticity, however, is also limited by multiple ecological factors, for example, extreme levels in a given abiotic factor can reduce plasticity to another factor (Valladares et al. 2007).
The traits present in a plant community also reflect the interplay between evolutionary and assembly processes and the physical environment (Sect. 20.3.2). Thus, understanding how and why plant traits vary among species and sites is a critical step towards understanding ecosystem properties and their functioning. Hence, trait-based ecology has received much attention over the last decade to acquire a better understanding of how traits influence species distribution, interactions and functions (e.g. Garnier et al. 2015 for a recent synopsis with extensive literature). Global data sets covering hundreds of traits and thousands of species are available (Kattge et al. 2011), and handbooks about measurements of traits are useful for standardisation and cross-study comparisons (e.g. Pérez-Harguindeguy et al. 2013).
Once traits and their respective associations with ecological responses or effects have been clarified, different measures of functional diversity can be determined. A very critical aspect for its determination is the need to select those traits that are relevant for the process or function of interest, which thus must be defined explicitly. In general, measures of functional diversity fall into two main classes: (1) discontinuous measures, that is, categorising species into plant functional groups or types, and (2) continuous measures, that is, measuring the spread of species in an n-dimensional trait space.
20.2.3.1 Plant Functional Groups
A rather broad measure of functional richness is that of the number of plant functional groups , also often referred to as plant functional types. Plant functional types are groups of species with similar suites of co-occurring functional attributes, such as comparable physiological behaviour (e.g. C3 and C4 plants) (Sect. 12.1), similar morphology or growth forms (e.g. stem succulents, lianas) (Table 20.3), temporal niches (e.g. spring geophytes, pluviotherophytes, early or late seasonal species) or similar dispersal syndromes (e.g. anemochory, zoochory) (Sect. 18.2). In addition, functional response groups and functional effect groups are respectively species that show a similar response to a particular environmental factor (e.g. sprouters, being plants that are able to resprout after fire) (Sect. 13.5) or that have similar effects on ecosystem processes (e.g. nitrogen fixers, which exert a significant influence on biogeochemistry). One major reason for reducing the huge variety of different organisms into such functional groups is the need to simplify floristic complexity for global vegetation models (Sect. 22.4), for vegetation mapping, and for monitoring purposes. Methodologically, such functional groups are identified via a priori knowledge about functional attributes of species (e.g. C3 and C4 pathways) or by cluster analyses of trait values.
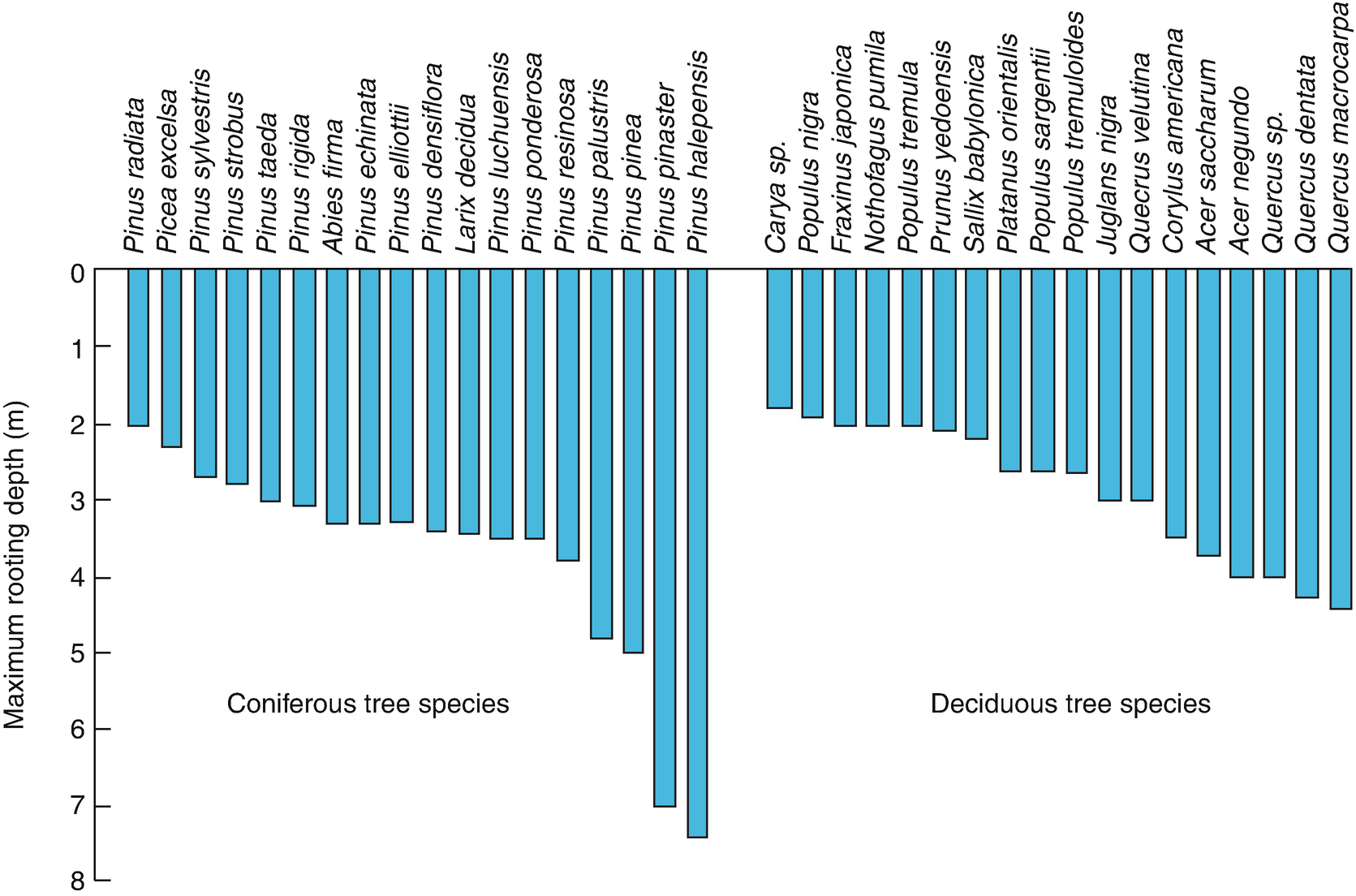

Effects of different species on soil nitrate availability. Species were grown in monocultures under identical environmental conditions, so that differences in soil nitrate availability are only due to ecological differences among species. Legumes (blue) fix nitrogen, but to varying degrees, resulting in higher nitrate values than under forbs (grey) and grasses (white). Phalaris: P. arundinacea, Leucanthemum: L. vulgare, Ranunculus: R. acris, Achillea: A. millefolium, Phleum: P. pratense, Festuca: F. ovina, Dactylis: D. glomerata, Rumex: R. acetosa, Lotus: L. corniculatus, T.: Trifolium. (Palmborg et al. 2005)
20.2.3.2 Continuous Indices of Functional Diversity
Much information is lost by any classification procedure because many traits are not categorical but continuous and plastic. In addition, using functional groups implies that species within those groups might be exchangeable or redundant when it comes to determining the effects of diversity on ecosystems (Sect. 20.4). Continuous measures of functional diversity , in contrast, capture the heterogeneity and variability of traits within a community. These measures can be calculated for single traits (e.g. asking the question whether the variability of leaf N concentration can better explain primary production than its mean value) or for multiple traits together (e.g. whether a higher or lower variability of leaf N concentration, cuticula thickness and LDMC better explains herbivory rates).

Geometrical presentation of functional diversity indices. Two traits define a two-dimensional functional space for a local community of ten species (dots). Species are plotted in this space according to their respective trait values, with symbol size proportional to their abundances. The functional diversity of a community is thus the distribution of species and of their abundances in this functional space. For each component of functional diversity, two contrasting communities are represented, with low a, b, c and high a′, b′, c′ index values. Functional richness a and a′ is the functional space occupied by the community, functional evenness b and b′ is the regularity in the distribution of species abundances in the functional space, and functional divergence c and c′ quantifies how species abundances diverge from the centre of the functional space (Mouillot et al. 2011)
Functional richness represents the volume of the functional trait space that is occupied by the species present in a community (Fig. 20.9a, a′). It therefore reflects the potentially used niche space by a community, that is, the hypervolume of a Hutchinsonian multidimensional niche . It can be used to test the hypothesis whether ecosystem properties depend on the size of the functional space covered. For example, it could be hypothesised that a community composed of species with very different rooting depths and plant heights (i.e. high functional richness) can take up more nutrients from the entire soil profile and capture more light, and hence produce more biomass, than a community composed of flat rooting species and small statured plants only (Fig. 20.38). The index is positively correlated with species richness, but communities with the same number of species may differ in functional richness if the traits are more similar in one community than in others.
Functional evenness measures the regularity in the distribution of species abundances in the occupied trait space, with high values representing a rather regular distribution of traits (Fig. 20.9b, b′). Functional evenness can also be linked to the utilisation of resources. Staying with the aforementioned example, it could be tested whether a community with an even distribution of rooting depths and plant heights (i.e. high functional evenness) is more productive than a community dominated by flat rooting small plants because the soil profile and the above-ground space are more evenly occupied and utilised, which could lead to higher nutrient uptake and light capture.
Functional divergence estimates the position of species within the trait space, for example, by quantifying how species abundances diverge from the centre of the functional space (Fig. 20.9c, c′). A high value means that very abundant species are very far from the centre of the trait space. In our example, it can be tested whether communities with large differences in rooting depths and heights (i.e. high functional divergence) may have a high degree of niche differentiation and low competition for resources (Sect. 20.4.9), potentially resulting in increased productivity, in comparison with communities dominated by species with small differences in these two traits.
20.2.4 Phylogenetic Diversity

Development of species richness and phylogenetic diversity through time. The black phylogeny represents a community or region with recent speciation events, and the blue phylogeny represents a situation with older speciation events. Both phylogenies ultimately produce the same number of species, but the accumulated branch lengths (i.e. phylogenetic diversity) in the blue phylogeny is much higher because of early diversification (Swenson 2011). Reproduced with permission from the Botanical Society of America
Phylogenetic diversity is an important aspect in studying the evolutionary processes that produce patterns of biodiversity and to understand the ecological interactions that determine species assemblage (Sect. 20.3). Being a surrogate of the functional structure of communities, it is also used to test hypotheses about the relationship between plant diversity and ecosystem functioning (Sect. 20.4). In addition, it is an important measure to define conservation priorities because communities with a high phylogenetic diversity represent a larger store of genetic diversity, available for adaptation and innovation. Conserving such communities with a higher priority than those with low phylogenetic diversity would therefore maximise future evolutionary options.
20.3 Environmental Controls of Biodiversity

Maps of global plant species richness. a Species richness based on empirical data from inventories and data on abiotic factors, including climate. The diversity zones (DZs) are grouped according to species numbers per 10,000 km2 (Barthlott et al. 2005). Reproduced with permission from the Deutsche Akademie der Naturforscher Leopoldina - Nationale Akademie der Wissenschaften. b Species richness derived from simulations based on growth-limiting climatic scenarios only. The values are categorised into nine groups: (1) <2%, (2) 2–4%, (3) 4–10%, (4) 10–20%, (5) 20–30%, (6) 30–40%, (7) 40–60%, (8) 60–80%, and (9) 80% of the maximum diversity value simulated. (Kleidon and Mooney 2000)
The following sections mainly describe patterns of plant species richness across environmental gradients and their underlying mechanisms. Clearly, biodiversity today is greatly changed by humans, for example, through land-use change and management, eutrophication or, increasingly, also by climate change. These aspects are described in more detail in Part V.
20.3.1 Latitudinal Gradients
Barthlott et al. (2005) presented a detailed world map of phytodiversity (of vascular plants) that shows the large spatial gradients in plant species richness on a global scale. Such maps can be produced by overlying information about the spatial distribution of single species, which is derived from herbarium records, taxon revisions or range maps. An alternative to such a taxon-based approach is the inventory-based approach where species richness values from thousands of floras, local checklists and regional species accounts are used to calculate species richness at a specific spatial grid resolution. Since the sampled areas differ in size, the richness values for this spatial pixel have to be standardised using an empirical species–area relationship (Sect. 18.4). Finally, non-sampled areas are interpolated using data on climate, vegetation types and geodiversity. In the map shown here (Fig. 20.11a), which uses a 100 × 100 km grid, 10 diversity zones are graded according to the number of species, from fewer than 100 to more than 5,000 per grid cell. The zones of lowest plant diversity are located in the Arctic tundra, the driest deserts (e.g. parts of the Sahara) and high alpine deserts (e.g. Tibetan upland). These regions are characterised by a lack of available ambient energy or humidity, limiting plant growth. An exception to this rule is the Namib Desert in southern Africa, which has a very long evolutionary history, high heterogeneity in topography and soils, and highly predictable rainfalls from fog in winter. The centres of highest phytodiversity are located in the humid tropics, including the Tropical East Andes, North Borneo and New Guinea, but also on the Atlantic coast of Brazil. Interestingly, not all tropical regions outnumber non-tropical ones: for instance, plant species richness in the Congo basin, where large areas are still rather undisturbed by humans, is comparable to that in Central European regions, which have been under long-term human influence. Other extra-tropical regions of high diversity are located at the Maritime Alps in France, the Caucasus or the Cape of South Africa.
Are these patterns similar for different plant life forms ? Trees, being important structural components of forest ecosystems and delivering many ecosystem goods and services, such as timber and non-timber products, are relatively well known in terms of their taxonomy and distribution. In addition, the increasing number of permanent forest inventory plots also allows for an upscaling of tree species richness to continental scales, assuming a relation between the number of species and the number of individuals of a defined region. Using standardised species lists with abundance data in such inventory plots in wet, moist and dry tropical forests, a recent study could show that the number of tropical tree species ranges between 40,000 and 53,000 in total, in contrast to only 124 tree species in temperate Europe (Slik et al. 2015)! The Neotropics and the Indo-Pacific region have very similar tree species richness (between approx. 18,600 and 24,800 species), while tropical forests of Africa are rather species poor, with only between 4,600 and 6,000 species. Thus, at least tropical trees indeed show spatial patterns similar to those shown for all vascular plants (Fig. 20.11).
So what factors are responsible for these spatial patterns? Kleidon and Mooney (2000) compared the map by Barthlott et al. (2005) with a map in which the global diversity of vascular plants was reconstructed on the basis of a climate model. Despite the different resolutions, both maps agree to a large extent (Fig. 20.11b). Obviously, climatic conditions can explain biodiversity at the global scale to a substantial degree. Climatic factors mostly constrain plant survival at the time of germination and during the development of young plants, and sufficient precipitation during those stages appears as a decisive factor: the smaller the number of days with favourable conditions for plant growth, the greater the constraints for growth and the less the diversity of species.

Patterns of plant diversity along latitudinal and climatic gradients. a geographical distribution of tree species in North America. The isolines connect points with rather similar species numbers (Currie 1991). Reproduced with permission from University of Chicago Press. b Species diversity correlates well with climatic factors, such as evapotranspiration, that change along the latitudinal gradient, as shown for all vascular plant species of North America. The number of vascular plant species is based on grid cells of 100 × 100 km. Modified from Mutke and Barthlott (2005). Reproduced with permission from The Royal Danish Academy
But is climate indeed the only driver of global, large-scale patterns in plant diversity? What other factors might also play a role? Such questions fall within the purview of macro-ecology . Several, not mutually exclusive, hypotheses have been formulated to explain the high plant diversity in the tropics and the decrease of diversity towards higher latitudes (see overviews by, for example, Huston 1994; Rosenzweig 1995; Hillebrand 2004; Clarke and Gaston 2006). Due to lower climatic fluctuations during the ice ages in the inner tropics, long-lasting favourable climatic conditions enabled long and undisturbed adaptation and specialisation . This can be illustrated by looking at the tree diversity data: as shown earlier, Africa harbours only approx. one-fourth of the diversity found in North America or the Indo-Pacific region, which cannot be explained solely by its smaller size and lower environmental variability. Rather, African forests were shrinking to small refugia areas during the Pleistocene, resulting in large species losses. Expanding to the current area, these forests must be repopulated from a depleted species pool, while forest area in the other two regions has not experienced similar shrinkages (Slik et al. 2015). Similarly, geologically old regions of the Earth are generally particularly rich in species because of their long history of evolution compared to geologically younger parts. Higher solar radiation and higher soil water availability at the equator, leading to increased evapotranspiration, result in increased annual productivity, which is the basis for many other organisms that can exploit this resource. A comparison between global maps of net primary production (Sect. 21.2, Fig. 21.4) with that of plant diversity (Fig. 20.11) intuitively shows that there might be underlying factors that positively influence both productivity and diversity. This climate-driven “energy–diversity hypothesis ” has attracted substantial interest and is now considered one of the most important drivers of those latitudinal gradients (Fig. 20.12). In addition, higher predation and pathogen load all year round in tropical regions can reduce the dominance of single species, enabling the coexistence of other species with lower abundance. Finally, the tropical regions also have a higher land-to-sea ratio than regions at high latitudes, so that terrestrial diversity should be higher based on the species–area relationship (Sect. 18.4). In contrast, climatic regions requiring specialised adaptation by organisms to harsh conditions, such as boreal forest or tundra biomes, are often relatively poor in plant species owing to strong environmental filtering (Sect. 20.3.4). For the same reasons, the number of plant species generally declines with altitude in high mountains, although the diversity in mountainous areas is higher than in lowlands if based on the available area for plant growth (Körner 2003). This has been explained by their geographical isolation and the high degree of topographic complexity and strong climatic gradients, leading to a high number of small-scale structures (habitats) in a given space, which allows many specialised species to coexist. In addition, lower human impacts and low-intensity management regimes may have led to high levels of biodiversity in mountainous areas.
20.3.2 Environmental Heterogeneity
The relation between environmental heterogeneity and species richness, as mentioned earlier in connection with alpine regions, has for a long time attracted many ecologists in the search for mechanisms driving gradients in species diversity. Almost 100 years ago, Thienemann (1920) formulated two “biocoenotic laws” stating that the more diverse the environmental conditions and the closer they correspond to the “biological optimum”, the larger the number of species, and vice versa. It has been argued that spatial environmental heterogeneity could promote species richness through three major mechanisms (Stein et al. 2014). The first mechanism is based on classical niche theory (Sect. 19.3): the available niche space (in a Hutchinsonian sense, defined as an n-dimensional hypervolume, with the dimensions being environmental conditions, resources and biotic factors) should become larger if environmental gradients become steeper, if the amount of habitat types and the number of resources available increases, or if the physical structure of habitats becomes more complex. More species can be packed into a larger niche space.

Small-scale temperature heterogeneity in alpine grasslands. a False colour image based on infrared thermography of surface temperatures on a NNW exposed slope at the Furka Pass in the Swiss Alps under full direct solar radiation (12–18 h, August). Dark blue represents cold (2 °C) and magenta hot (24 °C) surface temperature (Scherrer and Körner 2010). Reproduced with permission from John Wiley & Sons. b Seasonal temperature differences between surface and air temperature during daytime hours per group of temperature indicator values. The numbers in brackets indicate the number of plant species within the different indicator groups; significant differences are denoted by different letters (Scherrer and Körner 2011). Reproduced with permission from John Wiley & Sons

Positive relationship between environmental heterogeneity and species richness. Effects of environmental heterogeneity were analysed separately for different categories (land cover, vegetation, climate, soil, topography). Mean effect sizes (Fisher’s z) that are significantly larger than zero indicate positive relationships; lines show 95% confidence intervals. Different letters indicate significant differences among categories. Diamond and dashed lines represent the overall weighted mean effect. Numbers in parentheses give the respective numbers of studies/data points. All coefficients are different from zero at significance levels: ***p < 0.001, **p < 0.01, *p < 0.05. Modified from Stein et al. (2014). Reproduced with permission from John Wiley & Sons

Disturbances create “windows of opportunities” for species establishment. Species previously absent from a plant community are able to colonise a site after disturbances, such as fire. Saponaria ocymoides (pink flowers) and Isatis tinctoria (yellow) 4 years after a stand-replacing fire in the Swiss Alps (Leuk). (Photo: M. Scherer-Lorenzen)
In the examples presented in the previous sections, plant diversity is statistically treated as the response variable, while abiotic and biotic site factors (e.g. availability of light, water and nutrients; disturbance regimes; herbivory) or modulators (e.g. temperature, pH) represent the independent variables that determine or explain the distribution of plant species and their diversity (Fig. 20.1). In the following section, we want to present in more detail one example of such biodiversity research, which is important to understand when we later discuss the functional importance of plant diversity (Sect. 20.4).
20.3.3 Productivity —Species Richness Relationships
There are striking biogeographical patterns of plant species diversity and several hypotheses exist to explain those patterns (Sect. 20.3.1). According to the energy–diversity hypothesis , plant diversity correlates well with measures of productivity along latitudinal gradients. Is this relationship ubiquitous and observable at various spatial scales?

Positive linear relationship between above-ground primary productivity and plant species richness. This example comes from the Inner Mongolia region of the Eurasian steppe and shows the productivity–diversity relationship across different spatial scales, a at the level of the plant community (Stipa grandis association), b at the level of the vegetation type (typical steppe), and c at the level of the entire biome (by association type). The fitted lines represent statistically significant linear (solid line) and quadratic (dashed line) relationships between productivity and species richness (Bai et al. 2007). Reproduced with permission from John Wiley & Sons

Examples of hump-shaped relationship between productivity and plant species richness: a in British herb-dominated communities (Al-Mufti et al. 1977). Reproduced with permission from Blackwell Publishing Ltd.; b in South African Fynbos communities (Bond 1983); c in pre-alpine wet grasslands in Switzerland (Schmid 2002). Reproduced with permission from Elsevier Science Ltd.
Is the hump-shaped relationship ubiquitous? A meta-analysis by Mittelbach et al. (2001) showed that for vascular plants, hump-shaped relationships indeed dominate the observed patterns, especially at local scales or in studies that crossed community boundaries, that is, that included data from several different communities. A positive linear relationship was the second most observed pattern and has been reported mostly at continental to global scales. Interestingly, several studies showed that the area below the hump-shaped relationship is often filled with data points so that the hump-shaped line may be regarded as an upper envelope curve or border line, rather than a line of fitted average values (Fig. 20.17).

Conceptual model to explain the hump-shaped productivity–diversity relationship. The table summarises the main postulated mechanisms leading to the hump-shaped curve. The photos show some examples that are typical for the three parts of the hump. a Linaria alpina, a pioneer species colonising a heavily disturbed rock fan with minor amounts of mineral soil in the Swiss Alps. b Highly diverse, extensively managed subalpine meadow in the Swiss Alps, with intermediate levels of soil resource availability (low fertiliser input) and disturbance (cutting, grazing). c Rumex alpinus (foreground) dominates a patch in an alpine pasture, with high inputs of nutrients from resting livestock (“Lägerflur”). (Photos: M. Scherer-Lorenzen)


Experimentally testing the decrease of species richness under high productivity levels. The experiment involved manipulation of soil nutrient levels and understorey light conditions in grassland model ecosystems. The four treatment combinations were “control” (unfertilised, closed lights), “fertilisation” (fertilised, closed lights), “light” (unfertilised, open lights) and “fertilisation + light” (fertilised, open lights). A Experimental set-up; for illustration purposes only, two open lights and one closed light are shown in the same experimental unit, but they were installed in different treatments. B Above-ground biomass production a, light availability in the understorey b, and change in plant species richness c in response to the experimental treatments. Points denote treatment means, and the intervals show least significant differences (treatments with non-overlapping intervals are significantly different at p = 0.05). PAR: photosynthetically active radiation (Hautier et al. 2009). Reproduced with permission from AAAS
Despite the fact that the humped-shaped relationship between productivity and diversity has been documented often, controversy remains about the generality of this pattern, its dependence on spatial scale, the history of community assembly, measures of productivity or other methodological inconsistencies. For example, two large-scale, global studies that applied standardised sampling designs found either general support for the humped-shaped relationship in grassland communities (Fraser et al. 2015) or no significant relationship between peak above-ground live biomass and fine-scale plant species richness (Adler et al. 2011). The differences between both studies might be due to different statistical approaches or to the inclusion/exclusion of highly productive sites, which were found to be very low in species richness, thus strongly influencing the decreasing part of the hump in the Fraser et al. (2015) study. But despite these differences, both studies showed that the humped-back model has quite low explanatory power, even if the relationship as such remains significant. Rather, it seems that productivity and richness are both influenced by a multitude of factors and processes, such as nutrient supply rates, disturbance, habitat heterogeneity, assembly history or management. Thus, instead of focusing too narrowly on bivariate patterns such as the hump-shaped curve, future investigations should look into multivariate mechanisms controlling plant diversity.
20.3.4 Biodiversity, Assembly Rules and Environmental Filters
-
Distance of sink (local community) from source (regional species pool) and the size of the sink ecosystem. These aspects have also been integrated into the equilibrium theory of island biogeography (Sect. 18.4)
-
The structure and characteristics of the habitat (e.g. disturbance regime, nutrient status, water availability, resource heterogeneity) (Sect. 20.3)

Processes influencing species diversity. These processes range over different nested spatial and temporal scales. Environmental filters occur at several scales, but two are shown here for simplicity. (Ricklefs and Schluter 1993)
-
Species morphology and their adaptations, affecting habitat selection and survival (Sect. 2.1)
-
Biotic interactions, such as competition, predation, facilitation, mutualism (Sects. 19.3 and 19.4)
-
Population dynamics, including dispersal and biotal interchange, that is, the flow of species between adjacent regions (Sect. 18.2)
-
Evolutionary processes, including selection, genetic drift and speciation (Sect. 17.2)
-
Mass and stochastic extinctions

Community assembly through environmental filtering. Plant trait convergence with increasing harshness results in decreasing functional diversity in different alpine plant communities in the Swiss Alps, at Albula Pass (A: subalpine rock vegetation, B: alpine-subnival rock vegetation, C: subalpine scree vegetation, D: alpine-subnival scree vegetation, E: Carex firma grassland, F: Elyna myosuroides grassland, G: snowbeds, H: fountain vegetation, I: fen). The harshness index was calculated based on on-site measurements of microclimate (wind speed, air and soil temperatures) and soil variables (soil vs. stone content, soil moisture), as well as altitude (to represent other factors that correlate with altitude). Functional divergence (Sect. 20.2.3) was calculated with 16 traits (4 growth-form-related traits, 9 leaf traits, and 3 shoot traits). (Schmid 2007)
In contrast, high trait divergence (or trait dissimilarity) indicates that other ecological mechanisms are present that limit trait similarity, such as biotic filters imposed by competition. The concepts of limiting similarity and competitive exclusion and the related resource-ratio hypothesis (Sects. 17.3 and 19.3) state that two species that compete for the same resources—presumably due to the same trait combination—cannot coexist. That is, locally coexisting species should be functionally dissimilar to promote niche differentiation , which is one major mechanism allowing coexistence based on classical niche theory (Sect. 19.3). Thus, other factors may still allow for coexistence, such as local disturbances that diminish the competitive dominance of few species and promote regenerative mechanisms to exploit recruitment opportunities.
The study of factors driving plant community composition, their diversity and the patterns and mechanisms of trait divergence and convergence is a lively field of research. It shows that the driving factors, such as niche-related factors, environmental conditions and their heterogeneity, change along spatial scales: at small scales, niche- and soil-related variables more strongly determine community composition, while heterogeneity and disturbance-associated parameters as well as climatic factors prevail at larger scales. In general, trait convergence or divergence depends on a variety of factors, including the selection of traits or trait combinations, the spatial scale at which environmental filtering takes place, productivity or species richness.
20.4 Biodiversity and Ecosystem Functioning
Does biodiversity matter for the functioning of ecosystems ? In other words, does it make any difference to the processes within an ecosystem if there are many or only a few species? These are central questions that arise when looking at ecosystems that differ significantly in their biological richness but that have a similar basic set of energy, matter, and information fluxes (Sect. 13.2). For example, both tropical forests, with their overwhelming richness in their flora and fauna, and extremely species-poor systems, such as lichen communities in Antarctica (Box 20.3), fix carbon through photosynthesis of the plant compartment, and organic matter is decomposed by microorganisms into mineral components, which are partly taken up by the primary producers again. Although admittedly simple, this example shows that processes that are central to the functioning of ecosystems might be maintained by many or very few organisms, which prompts the question whether there is any relationship between biodiversity and ecosystem functioning. Related to our conceptual framework (Fig. 20.1), we are now dealing with the links between biodiversity and ecosystem properties and processes. The field of ecology related to that question has been termed functional biodiversity research, also sometimes called biodiversity-ecosystem function (BEF) research, to contrast it with classical approaches that study the factors and processes that determine species coexistence and dominance and, thus, the diversity of ecosystems (Sect. 20.3). One motivation behind this work is the search for fundamental ecological mechanisms that determine the properties and processes of ecosystems mediated by interactions among a diverse array of organisms. However, the answers to these questions are not only of pure academic interest but are relevant for human well-being: the accelerating loss of biodiversity (Sect. 23.5) may have profound effects on the way ecosystems operate (“ecosystem functioning”; Box 20.4 for definitions of relevant terms) and on the delivery of ecosystem services, that is, the benefits humans derive from ecosystems (Sect. 21.1).
Box 20.3: How Many Species Are Required for a Functional Ecosystem?

Crypto-endolithic ecosystems in arid valleys in Antarctica. a Schematic representation of this ecosystem and the important ecosystem functions and matter fluxes (Woodward 1993). b Photograph of fractured sandstone, showing black (masses of fungal/mycobiont hyphae with enclosed groups of algal/phycobiont cells), white (loose web of mycobiont filaments) and green (algal cells) zones of this ecosystem. Each zone is only about 1–4 mm. Southern Victoria Land in Antarctica, Linnaeus Terrace, Asgard Range. c Electron microscope image of phycobiont cells and mycobiont filaments growing in airspaces of rock, ×1000. (Photos with courtesy by E. I. Friedmann)
Box 20.4: Terms and Definitions Used in Functional Biodiversity Research
The terms ecosystem (or ecological) processes and properties, functions or functioning, and services are central to the concepts of functional biodiversity research. They are used in the following sense (compiled from Naeem 2002; Hooper et al. 2005; Millennium Ecosystem Assessment 2005; de Groot et al. 2010; Díaz and Cabido 2001; Hillebrand and Matthiessen 2009; Stachowicz 2001); Chap. 13.
Ecosystem processes : the physical, chemical and biological actions or events that link organisms and their environment, for example, primary production, water dynamics, nutrient cycling.
Ecosystem properties : the size of compartments; for example, pools of material such as standing biomass or soil organic matter.
Ecosystem functions : the capacity of ecosystems to provide services—directly and indirectly—underpinned by biophysical structures and processes. They can be seen as intermediates between processes and services. Often used in the same sense as ecosystem processes.
Ecosystem functioning : flow of energy and materials through the arrangement of biotic and abiotic components of an ecosystem, that is, including many ecosystem processes.
Ecosystem services : benefits people obtain from ecosystems, including provisioning services (e.g. food, fibre, biological resources), regulating services (e.g. erosion control, climate regulation, pollination) and cultural services (e.g. spiritual and religious, recreational, educational). In addition, supporting services enable the production of other services (e.g. soil formation, primary production, nutrient cycling). Sect. 21.1.
20.4.1 Species Identity and Dominance Effects on Ecosystem Processes
Species differ in their physical structure and biomass produced, as well as in the amounts and combinations of resources they use and in their chemical composition. Hence, different species can have largely divergent effects on ecosystem properties and processes (Sect. 19.2). Therefore, biodiversity effects on ecosystem functioning cannot be understood without proper acknowledgement of the identity of species and their specific physiological and morphological characteristics, that is, their functional effect traits (Sect. 20.2.3). Identity effects are due to the presence/absence of particular species within mixtures and are distinct from species composition effects, which distinguish the effects of different species combinations. Examples of species identity effects are numerous. Tree species, for instance, differ widely in their effects on soil development and nutrient supply. In a replicated experiment with 14 gymnosperm and angiosperm tree species, Reich et al. (2005) observed that 30 years after the establishment of single-species plots, differences in litter calcium concentrations resulted in profound changes in soil chemistry and fertility on initially the same soils. In addition, earthworm abundance and diversity were higher in those stands where tree species rich in calcium were grown.

Effects of morphological, physiological and life history traits on soil food webs and ecosystem processes. Positive feedbacks between the below-ground and above-ground systems in fertile ecosystems and negative ones in infertile systems (dashed arrows) result in strongly differing resource dynamics and species compositions (Modified from Díaz et al. 2009; Wardle et al. 2004; Cotrufo et al. 2013). The photographs show typical examples from productive (left) and unproductive (right) plant communities in the subalpine zone of the inner Alps of Switzerland (Simplon Pass). Both communities occur at the same altitude (2000 m a.s.l.) but strongly differ in water and nutrient supplies. The productive community dominated by large and fast-growing herbaceous species (Cicerbita alpina, bottom, and Adenostyles alliariae, top) obtains water surplus and high nutrient inputs from adjacent slopes. The unproductive community is situated on wind-exposed hilltops with flat soils and is composed of tiny dwarf shrubs from the Ericaceae family with small, often evergreen leaves (here mainly Arctostaphylus alpinus). Reproduced with permission from Oxford University Press and AAAS. (Photos: M. Scherer-Lorenzen)
What is the relevance of such species identity effects for the biodiversity–ecosystem functioning relationship? Changes in land use and anthropogenic alterations of climate, nutrient cycles or consumer presence alter not only the number of species but also their relative abundance and, thus, dominance or evenness (Box 20.1). The English plant ecologist Phil Grime included the knowledge of strong identity effects in his formulation of the “mass ratio hypothesis ” (Grime 1998), which states that resource dynamics and other aggregate processes in communities and ecosystems should be strongly controlled by the structural and functional traits of the most abundant, that is, most dominant, primary producer (Sect. 20.2.3). In many cases, ecosystems are indeed dominated by one or a few species, with other species having rather low abundances (“subordinate” species) or occurring infrequently and in very low abundances (“transient” species). Hence, he argued that dominant species should have the highest impact on ecological processes such as biomass production, carbon sequestration or nutrient cycling, while subordinate species will impact these processes only to a certain degree, and transient species will have only very minor effects. Thus, the effect of dominance comprises both an effect of the degree of dominance (i.e. abundance) and the identity (i.e. presence and value of functional traits) of the dominant species. According to this hypothesis, diversity should have rather additive effects weighted by the biomass of the species present, with mixtures intermediate in performance between the corresponding monocultures. For example, Rothe and Binkley (2001) reviewed the empirical evidence on the nutritional interactions of tree species grown in monoculture and in two-species mixtures, focusing on foliar nutrition, soil nutrient supply, rates of nutrient input and patterns of root distribution. They showed that additive effects were most common, with few antagonistic and synergistic effects. Antagonistic effects are found if mixture performance is lower than expected based on monoculture performance, indicating interference competition. Correspondingly, synergistic effects could arise from positive interactions among species, such as niche partitioning and resource-use complementarity, or facilitation, for example, by nitrogen fixation of some species that increase the overall N availability for the entire plant community (Sect. 20.4.9 and Box 20.5).
20.4.2 Biodiversity Effects on Biomass Production

Early hypotheses on the relationship between biodiversity and ecosystem functions. “Natural level” indicates the maximum level of diversity typical for the ecosystem under study; positions to the left of this point refer to biodiversity loss. The terminology of these early hypotheses is rarely used in current publications, but it has been important for the discussions and improvements of biodiversity–ecosystem functioning research
This overview is organised according to the scientific approaches that were adopted in the respective studies, that is, observational and experimental studies (Sects. 14.1 and 14.2), as well as modelling exercises. It focuses on plant species richness as the measure of biodiversity and on biomass production as an important ecological process. Effects on other ecosystem functions are presented in Sect. 20.4.3.
20.4.2.1 Observational Studies
Observational studies in natural plant communities to quantify the effects of plant diversity on ecosystem functioning can be divided into comparative studies and monitoring or sample surveys. These approaches are described in detail in Sect. 14.1.

Relationship between tree species richness and growth, and stability of growth, quantified in comparative studies. a Increase in basal area from 2008 to 2010 in relation to tree species richness of canopy trees and successional age in subtropical forests of China. Stand basal area is the total sum of the cross-sectional area of tree stems per hectare (Baruffol et al. 2013). b Stability of biomass growth from 1992 to 2011, quantified by measuring tree ring increments, in pure (Mono) and mixed (Mix) forest stands in Europe. Each open circle represents an individual species, while the filled circle represents the average stability across all species and regions. Red: Mediterranean-mixed forests in Spain; orange: thermophilous deciduous forests in Italy; dark green: beech forests in Germany; light green: mountainous beech forests in Romania; light blue: hemiboreal, mixed coniferous–deciduous forests in Poland; dark blue: boreal forests in Finland (Jucker et al. 2014). Reproduced with permission from John Wiley & Sons
Especially under a more applied aspect of timber production, a related question is whether tree diversity could also influence the stability of growth (Sect. 20.4.8). Also adopting a comparative approach, it has been shown that wood production can be more stable through time in mixed than in pure stands of very different forest types in Europe, ranging from boreal to Mediterranean forests (Fig. 20.25b). If growing in mixture, the different tree species reacted more differently to variable climatic conditions throughout the past 20 years of growth (asynchrony) and showed less temporal variation in growth through time, which resulted in overall higher stability with increasing tree species richness (Jucker et al. 2014).

Associations between tree species richness and biomass production form monitoring and sample surveys. a Forest Inventory data set from Mediterranean forests in Catalonia, Spain, with wood production (mean + SE) plotted against number of tree species per plot in deciduous (white bars), coniferous (grey) and sclerophyllous (blue) forests; total number of plots: n = 5069 (Vilà et al. 2007). Reproduced with permission from John Wiley & Sons. b Forest Inventory data sets from several countries, showing tree wood production (mean ± SE) in pairs of monospecific and mixed forests for different European forest types. The dashed line represents the line of unity, that is, values above this line indicate higher wood production in mixed than in pure stands, for each forest type. BE broadleaved evergreen (n = 10,399), TD thermophilous deciduous (n = 1,580), CM coniferous Mediterranean (n = 13,921), MD mesophytic deciduous (n = 6,548), FP floodplain (n = 205), AC alpine coniferous (n = 12,719), BH: boreal-hemiboreal (n = 3,019), NP non-riverine pioneer (n = 754), BC beech (n = 2,389), AO acidophilous oak (n = 119), EP exotic plantations (n = 3,612). After Vilà et al. (2013)
These examples show that trees growing together with many other different species are less negatively affected by interspecific competition than by intraspecific competition, presumably because of niche differentiation and resource-use complementarity , or facilitation among species, and can thus have larger growth. Such highly favourable conditions for growth in mixture may also increase resistance and resilience to perturbations or environmental fluctuations, so that trees may be able to remain productive under less optimal conditions. We will come back to these mechanisms of positive diversity effects in Sect. 20.4.9. However, there are also examples from other comparative studies that do not support these positive tree diversity effects on growth and stability and that rather suggest increased competition in mixtures than in monocultures. These contrasting views may depend on the forest type and its management; for example, silvicultural thinning to reach an optimised tree density for timber may mask any potential diversity effects on growth. Opposite patterns of the diversity–productivity relationship found in natural ecosystems may also be due to different environmental contexts, which is discussed in detail in Sect. 20.4.7. In addition, processes that favour species similarity through strong environmental filtering (e.g. harsh climatic conditions, enabling only few species with similar adaptations to persist) (Sect. 20.3.4) should result in rather low or even absent effects of plant species on ecosystem functions because diversity effects are essentially based on differences among species in functional effect traits (Sect. 20.2.3 and Fig. 20.1). However, processes that limit similarity (e.g. competition for the same resources, effects of shared pathogens) and that enable species coexistence may result in strong diversity effects, which should thus be rather frequent in natural ecosystems.
Despite these opposing views and the inherent limitations concerning causality of observed associations between diversity and function in observational studies (because both diversity and functions can be influenced by the same environmental factor) (Sect. 14.2), there is now increasing evidence that suggests that plant species richness is an important predictor of ecosystem functioning in natural plant communities, at least in forests. The scatter in the shown data sets nicely demonstrates that additional abiotic and biotic factors influence ecosystem functions, such as climatic or soil variables, and management. In addition, identity effects are usually very prominent in observational studies, that is, ecosystem functions are often strongly determined by the dominant species (Sect. 20.4.1). Thus, ecosystem ecology and the search for biodiversity effects on ecological functions cannot be tackled as univariate problems, but a multitude of abiotic and biotic factors need to be taken into account that interact in complex ways. Another way to reduce the effects of confounding factors is the use of experimental approaches.
20.4.2.2 Experimental Studies
Experimental studies in biodiversity–ecosystem functioning research aim to decouple biodiversity from environmental conditions in order to identify causality and mechanisms of diversity effects. Thus, the level of plant diversity is manipulated experimentally by researchers. These experiments can be done in natural communities (removal experiments) or with artificially created communities (synthetic assemblage experiments or simply biodiversity experiments). These approaches are described in detail in Sect. 14.2.
Of the two experimental approaches to studying biodiversity–functioning relations, removal experiments are highly underrepresented, although they can be useful to understand the ecosystem consequences of local, non-random extinctions, changes in natural abundance and complex interspecific interactions. For example, Wardle and Zackrisson (2005) removed plant species and entire plant functional groups from the understorey vegetation of 30 forested islands in northern Sweden. These islands differed in size, leading to a successional gradient from early successional, highly productive to late successional, less productive systems. Losses of functional groups and species decreased key ecosystem processes such as biomass production, litter decomposition and soil respiration. However, removals had strong effects on some islands but not on others, depending on island size and thus successional status and resource dynamics. This example therefore nicely highlights the importance of the specific context of study systems and shows that results cannot necessarily be generalised to other systems until properly tested.
-
Naeem et al. (1994, 1995) designed artificial ecosystems (“Ecotrons”) with varying species richness levels of plants, herbivores and decomposers in climate chambers. The medium- and low-diversity communities were a subset of high-diversity communities, resembling functionally depauperate descendants. Only identical species mixtures were replicated at each level of diversity. Thus, the effect of the presence of highly productive species only occurring at higher diversity levels could not be separated from richness effects. Towards the end of the experiment, high-diversity communities had lower soil nutrient concentrations and were consistently more productive than medium- and low-diversity treatments
-
Tilman and colleagues conducted several large-scale experiments at Cedar Creek, Minnesota, that showed that soil nitrate concentrations within and below the rooting zone decreased with increasing numbers of plant species, while biomass production increased (Tilman et al. 1997a, 2001). Interestingly, many mixtures had higher biomass than the single best performing monoculture (“transgressive overyielding ”), refuting the sampling hypothesis as the sole explanation of the results and indicating complementarity effects (Sect. 20.4.9). On average, the diversity effects became stronger over time, due to increasing use of the available niche space (i.e. complementarity among co-occurring species). This resulted in a shift from asymptotic diversity–productivity relationships in young, developing communities to more linear relationships in mature, fully developed communities (Reich et al. 2012)
-
Within the pan-European project BIODEPTH, the same basic design with manipulation of plant species richness and number of functional groups was replicated at eight different sites across Europe (Sweden to Greece). Across all sites, soil mineral nitrogen concentrations decreased with the number of functional groups (but not with plant species richness) (Spehn et al. 2005), and at the German site nitrate leaching also decreased significantly with both measures of diversity in the presence of N-fixing legumes (Scherer-Lorenzen et al. 2003). Above-ground biomass production increased with species and functional group richness (Hector et al. 1999). This effect got stronger over time and was mainly driven by complementarity, although sampling effects also contributed to the observed results. Unfortunately, not all species were grown as monocultures at some sites, which is necessary to unambiguously test for sampling effects. The biomass results also strongly differed among sites, with very large positive effects in Germany, Switzerland, Sweden, Portugal and the UK (Sheffield), unimodal or idiosyncratic relationships in Ireland and UK (Silwood) and no effects in Greece
-
The Jena Experiment in Germany yielded results very similar to those described earlier: soil nutrient concentrations decreased with increasing plant species richness, or number of functional groups (grasses, small and tall herbs, and N-fixing legumes) (Oelmann et al. 2007). In parallel, biomass production increased with increasing diversity, both above and below-ground. The slopes of these relationships increased over the years, suggesting stronger and sustainable diversity effects with time. Again, complementarity effects prevailed, and the diversity-driven increase of biomass was a result of increasing plant density rather than plant size (Marquard et al. 2009)

Results from selected classical biodiversity experiments adopting the “synthetic assemblage” approach. Data showing plant diversity effects on the efficiency by which communities take up limiting resources (light and nutrients) and convert these into biomass (productivity). A: Naeem et al.’s (1994) experiment in the climate chamber Ecotron facility at Imperial College, UK. Shown are a the temporal evolution of ammonium concentrations in the soil, which showed no clear pattern with diversity levels, and b the standing above-ground biomass (circles: means and standard errors by diversity level; points: values for each experimental assemblage (Naeem et al. 1995). Reproduced with permission from the Royal Society of London. (Photo P. Manning) B: The Biodiversity II field experiment at Cedar Creek, Minnesota, USA, with plant species richness effects on a soil nitrate concentrations (Tilman et al. 2002) and b standing above-ground biomass (Tilman et al. 2001). Reproduced with permission from AAAS. A variety of differently designed biodiversity experiments have been established at that site. (Photo J. Miller) C: The BIODEPTH experiment replicated at eight European sites. Shown are a values for nitrate loss to the groundwater at the German site (Scherer-Lorenzen et al. 2003; reproduced with permission from John Wiley & Sons) and b biomass production across all sites (Hector et al. 1999; reproduced with permission from AAAS) in relation to plant species richness. (Photo M. Scherer-Lorenzen) D: The Jena experiment in Germany, with plant species richness effects on a soil nitrate pool sizes (Oelmann et al. 2007; reproduced with permission from the Soil Science Society of America) and b biomass production (Ravenek et al. 2014). (Photo: Forschergruppe Jena Experiment)

Summary of results from several biodiversity experiments on key ecosystem functions. Of those studies that have shown significant plant diversity effects on ecosystems, most have found an asymptotic relationship between the number of plant species (producer richness) and three important ecosystem processes a: plant biomass production, b: plant nutrient uptake, c: plant litter decomposition). Fewer studies reported linear relationships or those that correspond to the loss of keystone species, where such losses lead to large declines in functioning. Given are the number and percentages of the studies analysed by Cardinale et al. (2011). Reproduced with permission from the Botanical Society of America
20.4.2.3 Modelling Studies
Biodiversity–ecosystem functioning research can also be done solely on the computer, that is, in modelling studies, permitting the examination of specific mechanisms or the extension to larger spatial scales and longer time durations than empirical work. Models have been used in functional biodiversity research since early on and helped greatly in sharpening the hypotheses of underlying mechanisms. The first modelling exercises that explicitly dealt with biodiversity effects on ecosystem functioning mainly confirmed the observed positive diversity effects on productivity or nutrient retention found in experimental studies. For example, Tilman et al. (1997b) used models of interspecific competition for nutrients and randomly assembled communities with different species numbers. All species were identical, except that they differed in their R* values, that is, the concentration to which a resource is reduced by an equilibrial monoculture of that species (Sect. 17.3 and Box 19.2). They could show that, on average, productivity increases asymptotically with plant species richness, irrespective of the model complexity (with competition for one or two resources or with a generalised niche model). At the same time, nutrient retention also increased, which corresponds well to experimental results. This suggests lower nutrient losses at higher levels of diversity, for example, through soil nitrate leaching. In summary, the study showed that variation in species traits (R*, and the size of the niche spaced covered by each species) is sufficient to establish a positive richness–productivity relationship based on fundamental mechanisms of interspecific competition. Greater variation in species traits is magnified by interspecific interactions, which cause the average response of multispecies communities to differ from the response of the average monoculture. Both complementarity and selection effects (Sect. 20.4.9 and Fig. 20.37) contribute to this effect. Other models also confirmed the hypothesis that plant diversity can exert effects on ecosystem functions, for example, mechanistic models in spatially structured ecosystems in which plants compete for a limiting soil nutrient (Loreau 1998) or models of recruitment limitation in heterogeneous habitats or competition–colonisation trade-off models (Mouquet et al. 2002). However, these models also reveal situations where no diversity–function relationship should be expected, for instance, when species are redundant in their functional role (e.g. occupying the same spatial niche) and when the average resource-use intensity of the species is not affected by species diversity (which can hardly be found in real situations). These models also show that the slope of the diversity–function relationship depends on the mechanisms of species coexistence and on the causes of species richness gradients.

Modelled productivity responses to changing tree species richness. a Estimates of productivity against original species richness for 11 different sites in Switzerland, for which the model has been parameterised. Shaded areas between dashed lines represent a 95% confidence interval of the true median of productivity. Similar results were obtained with realised species richness after 2000 years of simulated forest dynamics. b Hypothetical mechanisms underlying the observed positive diversity effect on productivity, which can be divided into two categories: those affecting the mean values of some forest characteristics (state) and those affecting the variability of these characteristics (variability). The numbers in brackets refer to the detailed explanations in the text (Morin et al. 2011). Reproduced with permission from John Wiley & Sons
20.4.3 Biodiversity Effects on Other Ecosystem Functions
The first experiments quantified biodiversity effects on the basis of a few ecosystem functions only—mainly measures of productivity and of resource uptake, as exemplified in the previous section. Later experiments often simultaneously measured a large variety of ecological properties and functions, including, for example, litter decomposition, carbon sequestration, soil water and nutrient dynamics, or greenhouse gas emissions. Several of these response variables are also affected by plant species composition and diversity, and some examples are presented here.

Examples of plant species richness effects on soil ecosystem functions. All examples are from the Jena Experiment, Germany. a Porosity or infiltration capacity increases significantly with plant diversity, suggesting improved soil hydrological properties in mixtures (Fischer et al. 2015). b Changes in soil carbon stocks (calculated as the product of soil bulk density and its carbon concentration) between the beginning of the Jena Experiment (2002) and 9 years later (2011) significantly increase with plant species richness, in communities both with legumes (triangles) and without legumes (circles), implying greater carbon storage with increasing plant diversity (Lange et al. 2015). Since soil bulk density decreases with increasing diversity in this experiment (inverse pattern of porosity, see panel a, the rise in carbon storage is largely driven by changes in carbon concentrations, for example, through increased root exudation and microbial biomass. c Microbial biomass significantly increases with plant species richness only after some years after the establishment of new plant communities. Different letters indicate significant differences (Eisenhauer et al. 2010). Reproduced with permission from John Wiley & Sons
In biodiversity experiments, soil -related functions often react with some time lag after the initiation of the experiments in comparison with those above-ground, as seen for microbial biomass, for instance (Fig. 20.30c). Most plant diversity experiments following the synthetic community approach involve some land-use change and pretreatments or soil disturbances, such as ploughing, harrowing or herbicide application, before new communities are established. Many soil organisms therefore suffer a decline in abundance. Only with ongoing succession and recolonisation by soil organisms may differences in plant community composition and diversity finally manifest in specific soil communities and functions (Eisenhauer et al. 2010; Sects. 13.4 and 14.2). Such findings also highlight the need to run biodiversity experiments for prolonged periods of time.
20.4.4 Biodiversity Effects on Other Trophic Levels

Plant diversity begets diversity of other organismic groups. All response variables are scaled to [0, 1], and every curve is fitted using a power function with covariates. Identical colours in each horizontal pair of panels indicate identical groups of organisms. Invaders are non-sown plant species (Scherber et al. 2010)

Associational resistance and susceptibility. a Vole damage and moose browsing in a Finnish tree diversity experiment. Voles restricted their feeding to only the most palatable tree species, supporting the associational resistance hypothesis. In contrast, moose feed on more varied tree species, suggesting associational susceptibility (Vehviläinen and Koricheva 2006). b Pathogen load by mildew species growing on oaks decreases with the diversity of local tree neighbourhoods, that is, the number and abundance of tree species surrounding a target tree, representing a case for associational resistance. (Hantsch et al. 2013)
20.4.5 Biodiversity and Multifunctionality

Proportion of species needed to support ecosystem functioning. If multiple contexts are considered, more species are needed to promote ecosystem functioning, for example, when more years a, places b, ecosystem functions c or environmental change scenarios d are taken into account. The “proportion of species promoting ecosystem functioning” results from modelled ecosystem functioning response variables (e.g. productivity, litter decomposition) as a function of the presence or absence of each study species at the plot level and is a relative measure of the number of species needed. The dashed blue line represents the grand mean fitted across all studies; the solid blue lines represent the response found in single studies; box plots summarise observed data from the 17 grassland biodiversity experiments included in the study. (Isbell et al. 2011)
20.4.6 Different Metrics of Biodiversity Affecting Ecosystem Functioning
As exemplified by the selection of the studies discussed earlier, there is now substantial evidence that biomass production and many other ecosystem functions increase with increasing plant species richness, that is, taxonomic diversity. Does this pattern also hold true if other metrics (evenness) or facets of biodiversity (genetic, phylogenetic, functional or structural) are considered?
Increasing evenness of plant communities, that is, how evenly the abundance or biomass is distributed among species, commonly results in higher levels of biomass production. Starting with the experiments of Wilsey and Potvin (2000) in oldfields of Quebec, Canada, several studies have now confirmed their results, while others reported increased productivity with increasing dominance of species (Hillebrand et al. 2008). These contrasting results can be explained theoretically by the prevalence of species interactions influencing ecosystem processes: in the case of synergistic interactions, such as complementarity or facilitation (based on niche differentiation among species) (Sect. 20.4.9), increasing evenness should result in higher process rates, and increasing dominance to lower rates. In contrast, if species identity (i.e. the dominant trait) predetermines processes (corresponding to selection or sampling effects) (Sect. 20.4.9), these processes should be largely influenced by increasing dominance. In addition, under longer time scales with fluctuating environmental conditions, a higher evenness should allow communities to adapt to new ecological conditions and sustain ecosystem process rates due to higher trait variance. In contrast, under stable conditions, a certain optimal trait configuration should result in the highest process rates, and any deviation from this trait configuration through increasing evenness would reduce process rates.
Experiments that created a gradient of plant genetic diversity within species, for example, by planting communities with different numbers and combinations of genotypes (e.g. of seagrass (Zostera marina), wheat (Triticum aestivum), wall cress (Arabidopsis thaliana), evening primrose (Oenothera biennis) or different grassland species) also showed positive diversity–productivity relationships, often in the same magnitude as those found with species diversity (e.g. Zeller et al. 2012). Genetic diversity seems to be especially important for the temporal stability of biomass production (Prieto et al. 2015). As mechanisms, complementarity in resource use and facilitation (Sect. 20.4.9) in those mixtures of genotypes that differ in functional traits have been suggested.
Manipulating phylogenetic diversity, that is, planting communities differing in the evolutionary relatedness of the constituting species (Sect. 20.2.3), also often leads to similar results; for instance, Cadotte (2013) showed that if species were grown together with close relatives (low phylogenetic diversity) the biomass production was similar to that in monoculture. If they were grown with distantly related species (i.e. high phylogenetic diversity), they produced more biomass than expected from their monocultures. Thus, distantly related species differ more strongly in their niche requirements than closely related species, leading to an occupation of different niches that allows resource-use complementarity (Sect. 20.4.9).

Aerial view of a trait-based tree diversity experiment (BIOTREE-FD). The experiment consists of stands differing in functional diversity, with the number of species kept constant at a level of four per plot. The species pool consists of 16 European temperate tree species. Within each hexagonally shaped plot, species were planted in round monospecific patches to delay outcompetition of slow-growing species in the early years (Scherer-Lorenzen et al. 2007). (Photo: K. Kovach)
In analogy to the experimental manipulation of compositional or functional diversity, one might also think of biodiversity experiments that create a gradient of structural diversity , β-diversity or even landscape diversity directly, but this has not been done so far. It would be worth doing if we wish to understand the multiple effects that different facets of biodiversity may have on ecosystem multifunctionality, and across multiple spatial scales. Indeed, data from observational studies indicate that growth can be positively affected by increasing structural diversity in forest stands (Lei et al. 2009).
Although it has often been claimed that one biodiversity metric (e.g. functional diversity) should be better suited to explain diversity effects on ecosystems than others (e.g. species richness), the choice of the metric will certainly depend on the research question and on the taxa to be investigated.
20.4.7 Context Dependency of Biodiversity Effects on Ecosystems
-
The values of ecosystem processes could increase or decrease with changing environmental conditions, but similarly across the entire range of diversity. In statistical terms, the slope of the relation does not change, only its intercept, and the interaction term between diversity and environmental conditions will be non-significant (Fig. 20.35a). As an example, drought stress reduced biomass production at all levels of plant species richness by the same absolute value in the Jena Experiment (Vogel et al. 2012).
-
The values of ecosystem processes change under different environmental conditions, but the magnitude of this change depends on plant diversity, leading to changing slopes of the diversity–function relationship, and a significant diversity by environment interaction. If the slope increases, that is, when diversity effects become stronger under a changed environment relative to a control situation, two possibilities exist (Fig. 20.35b):
-
Plant diversity effects are stronger under more favourable conditions, that is, when resources are plentiful; examples include a larger soil volume that can be exploited or higher nutrient availability after fertilisation. This biotope-space hypothesis postulates that the niche space of individual species can expand into a larger biotope space and that more species with different niche requirements can be “packed” into the niche space under more favourable conditions (Dimitrakopoulos and Schmid 2004). Therefore, resource-use complementarity can be greater and beneficial biodiversity effects should increase under favourable conditions, where high soil resource availability is hardly limiting growth. For example, diversity effects increased with fertility of the soil in an experiment with annual plant communities and NPK fertilisation (Fridley 2002).
-
Plant diversity effects are stronger under harsh environmental conditions because they often rely on facilitation or positive interactions among species (Sect. 20.4.9), which are more pronounced under harsh conditions: according to the stress-gradient hypothesis , interactions among coexisting species shift from negative (competition) to positive (facilitation) as environmental stress increases from favourable to harsh conditions (Callaway 2007). For example, in a study with freshwater marsh plants, the absolute values of biomass production decreased in more stressful environments (drought, salt, shade) relative to a control treatment, but diversity effects increased (Steudel et al. 2011). Comparative studies from natural and semi-natural ecosystems, for example, forests, mostly support the view that diversity effects become stronger under harsh conditions.
-

Context dependency of biodiversity–ecosystem function relationships. a The slope of the relationship between plant diversity and ecosystem function stays constant with environmental context, but the absolute values of the response variable are different depending on environmental conditions. The example shows plant diversity effects on above-ground biomass under control conditions (black circles, solid line) and under drought, simulated with rainout shelters (open circles, dotted regression line) (Vogel et al. 2012). b The slope of the relationship between plant diversity and ecosystem function changes with environmental context, with stronger diversity effects under either favourable conditions (left example: diversity effects on above-ground biomass under different soil fertility levels, Fridley 2002) or stressful conditions (right example: diversity effects under control and drought, salt, or shade treatments) (Steudel et al. 2011). Reproduced with permission from John Wiley & Sons
Support for both scenarios has been documented, so it is not possible to say whether diversity effects should generally be stronger under harsh or favourable conditions. Presumably, the type of environmental conditions, that is, which resources limit plant growth most, and the nature of species interactions might both be crucial for the slope of the diversity–function relationship under different ecological contexts. For example, imagine a gradient of growing conditions, ranging from low supply of soil resources, where nutrient or water availability limits plant growth, to high soil resource availability, where light is the major growth-limiting factor. If species interactions result in improved light absorption, for example, through canopy stratification, or higher light-use efficiency, then the diversity effects should be stronger under more favourable conditions, in line with the biotope-space hypothesis. In contrast, if species interactions result in greater soil resource availability, for example, through N-fixation or accelerated rates of nutrient cycling, the diversity effects should increase with decreasing growing conditions, based on the stress-gradient hypothesis. Thus, the strength of positive interactions among species (complementarity , facilitation ) will change along the gradient of growing conditions (Forrester 2014).
20.4.8 Plant Biodiversity and the Stability of Ecosystem Functioning
A long-standing hypothesis in ecology is that species diversity is positively related to ecosystem stability, which dates back to the seminal work of Charles S. Elton (1958) and which has fascinated ecologists ever since. An exhaustive overview of this “diversity-stability hypothesis” can be found in Griffin et al. (2009), Loreau and Mazancourt (2013) and Oliver et al. (2015). Interestingly, this field of ecology has been largely theory-driven. Early theory predicted that more diverse communities and food webs were more stable and more resistant to invasion by other species (e.g. MacArthur 1955). Later, Lotka-Volterra models of interspecific competition showed that communities became less stable as the number of interacting species increased (May 1973). In contrast, future statistical models showed that diversity may buffer environmental fluctuations through the scaling relationship between the mean and the variance (statistical averaging, or the so-called “portfolio effect ”), even without interactions among species (Doak et al. 1998). This portfolio effect compares the temporal stability of ecosystem functions with that of financial assets, which show lower fluctuations and, thus, higher stability when partitioned across multiple stocks. Statistical averaging can also result in a positive diversity–stability relationship in the presence of species-specific responses to changing environmental conditions and functional redundancy within groups of species (“insurance hypothesis”) (Yachi and Loreau 1999). Imagine that species differ in their requirements for abiotic or biotic environmental factors (e.g. temperature, soil moisture, nutrient availability), that is, in their fundamental niches. These species will respond to environmental fluctuations not similarly, but asynchronically, and declines in some species or populations will be compensated by increases in others, resulting in less variation in process rates at the aggregate community or ecosystem levels or in no net change of ecosystem functioning, a phenomenon called compensatory dynamics . Thus, a high diversity of species’ responses within functional groups further stabilises ecosystem functions through the asynchrony of species responses to environmental fluctuations . This mechanism can be seen as a form of temporal complementarity between coexisting species. Plant diversity can also increase the temporal stability of biomass production if mixed communities produce more biomass than monocultures (“overyielding ”) (Sect. 20.4.9) while keeping temporal variability constant.

Plant biodiversity effects on different aspects of ecosystem stability. Ecosystem stability is defined as low temporal variability a, resistance b or resilience c of biomass production. As perturbations, severe dry or wet years were considered across 46 plant diversity experiments. Thin lines represent mixed-effect model fits for each study a or a climatic event within each study b, c. Thick lines are model fits across climatic events and studies with 95% confidence interval bands. Axes are logarithmic. (Isbell et al. 2015)
20.4.9 Mechanisms Underlying Biodiversity–Ecosystem Functioning Relationships
Based on hundreds of observational and experimental studies, there is now broad consensus that plant diversity affects many ecosystem properties and processes, irrespective of the metric of biodiversity used. More diverse systems consistently have higher biomass production, higher nutrient uptake and, consequently, lower leaching losses to groundwater, among other effects. What are the underlying mechanisms that could explain such biodiversity–ecosystem function relationships?
In general, trait differences among species are the underlying causes of any biodiversity effects on ecosystem functioning . In the debate about the relevance of results from manipulative experiments, often a distinction was made between the effects of species numbers and of species identities (i.e. their functional traits). Many studies have shown that the identity of species within a mixture is more important than the number of species. However, because no two species are identical in their characteristics (e.g. growth rate, size, nutrient use efficiency, leaf lifespan), it is impossible to manipulate species richness without selecting species with different characteristics. Thus, it becomes clear that there is no magic effect of numbers of species per se and that any effect will arise from functional differences between species and from species interactions. There can not be any relationship between species richness and ecosystem processes without these functional differences between species. All theoretical models of diversity–functioning relationships also include assumptions concerning differences in species’ traits, and there is no theoretical mechanism that would produce any relationship between species richness and ecosystem processes with identical species. Therefore, the characteristic traits of species and, thus, the diversity of functions these species perform are important determinants of ecosystem processes.
-
Sampling and selection effects.
-
Niche differentiation and resource-use complementarity.
-
Facilitation and mutualisms.
-
Trophic interactions and negative density-dependent effects.
20.4.9.1 Sampling and Selection Effects

Theoretical models of selection and complementarity effect. a Selection effect. Note the upper flat bound of the point cloud (dashed line), which indicates that the productivity of highly diverse mixtures is not higher than the single best monoculture. b Complementarity effect. Note the increasing upper bound of the point cloud (dashed line), which indicates that the productivity of highly diverse mixtures is higher than the single best monoculture. Triangles denote results from different simulation runs with different species compositions; lines and large dots show the means (Modified from Tilman et al. 1997b). Reproduced with permission of the National Academy of Sciences, USA
20.4.9.2 Niche Differentiation and Resource-Use Partitioning : The Complementarity Effect
Resource-based competition theory predicts the coexistence of species if interspecific competition is lower than intraspecific competition . This could be achieved by differences in species’ requirements for abiotic or biotic resources, habitat or environmental conditions, and, hence, in their niche space. Niche differentiation allows more species to coexist, that is, it promotes diversity (Sect. 19.3). Since plants depend on a small and common set of abiotic (water, light, CO2, mineral nutrients) or biotic resources (pollinators, mycorrhiza, among others), partitioning of resource niches in plant communities is less obvious than in animal communities. One way in which plants could differ in resource niches is by partitioning a common resource in space (e.g. access to nutrients in different soil layers due to differences in rooting depths), time (e.g. different nutrient uptake phenology), chemical form (e.g. nitrogen nutrition via nitrate, ammonium or organic N) or a combination thereof.
Such partitioning of the available resource pool among the coexisting species allows them to use resources in a complementary way. Hence, a combination of species could obtain more resources than could any species living by itself, that is, the per capita performance of species in species-rich communities is higher than in species-poor ones. Thus, niche differentiation or resource partitioning and the resulting resource-use complementarity can lead to increased yield in mixtures compared to those of the corresponding monocultures due to a larger available total resource pool.
The principle of resource complementarity has been known for a long time. The German silviculturalist Heinrich Cotta (1763–1844) argued against large-scale afforestation with fast-growing species in monoculture, which were established on the recently developed concept of sustainability by von Carlowitz (1713): “Endeavours to establish pure stands everywhere are based on an old and highly detrimental prejudice. […] Since not all tree species utilise resources in the same manner, growth is more lively in mixed stands and neither insects nor storms can do as much damage; also, a wider range of timber will be available everywhere to satisfy different demands…” (Cotta 1828, p. 115; translation by Pretzsch 2005). He thus recognised the fact that different tree species occupy different resource niches and that this results in higher yields and even greater stability against biotic or abiotic perturbations; he also somehow anticipated the concept of ecosystem services by relating this ecological principle to human demands. Later, Charles Darwin in The Origin of Species used the term “ecological division of labour” to describe ecological niche differences that will result in higher productivity in mixed plant communities (Hector and Hooper 2002): “It has been experimentally proved that if a plot of ground be sown with one species of grass, and a similar plot be sown with several distinct genera of grasses, a greater number of plants and a greater weight of dry herbage can thus be raised” (Darwin 1872, p. 113). Since then, the concept of resource complementarity has already been successfully used for a long time in intercropping or agroforestry to increase yields per unit land area, for instance. In these cases, species with markedly distinct functional properties are chosen to produce higher yields in mixtures than in monoculture, a phenomenon known as “overyielding ”.

Niche occupation and resource complementarity. The complementarity effect is conceptually related to niche differentiation along different resource axes (e.g. above- and below-ground space), with diverging consequences for species’ niche breadth, niche overlap among coexisting species and total niche breadth of the community (von Felten et al. 2009). a Species exhibit their fundamental niche (coloured boxes) if growing in monoculture, which are spread over the multidimensional niche space. b A gradient of plant species richness, for which the consequences are depicted for niche overlap and niche breadth c–e. Note that plant density remains constant, which is realised in biodiversity experiments using a replacement series or substitutive design (Box 19.1). c If one would combine the fundamental niche of several species along a gradient of plant diversity, niche overlap between species (grey areas) would be large. d Plants growing in mixtures are adjusting their niche space to avoid competition (realised niche), resulting in a smaller niche space of each individual species (cf. size of coloured boxes in c and d or of light green box in monoculture and in mixtures) and in lower niche overlap (compare grey areas in c and d). The lower niche overlap among species allows them to partition resources. e The total niche breadth, that is, the total volume occupied by coexisting species in the n-dimensional niche space, increases with the number of species present (total bar size). Each species contributes to total resource use (coloured stacked bars)
Typical examples of resource-use complementarity are mixtures of small and large statured plants, of plants with shallow and deep roots, of sun-adapted trees in the overstorey and shade-tolerant species in the understorey, of early successional and late successional species, of warm-season and cold-season grasses, or of nitrate- vs. ammonium-preferring species. In agroforestry and intercropping, such species have also been called species with good “ecological combining abilities” because they face less competition in combination with other species than when growing only with conspecific neighbours.
Box 20.5: Distinguishing Between Selection and Complementarity Effects
Discussions about the interpretation of early biodiversity experiments mainly centred on the question whether the observed patterns could be explained by niche complementarity or by the selection effect. It is now clear that these two classes of mechanisms are not mutually exclusive and that they can occur simultaneously. In addition, in most experiments, selection effects prevail during the early phases owing to the exponential growth of species that develop quickly and dominate mixed plots initially. Later in the course of the experiment, competitive dynamics take over and niche complementarity effects become stronger (Reich et al. 2012).

Net effects of species interactions. Depending on the type of species interactions, community-level ecosystem functions, such as biomass production, can be lower, similar or higher than expected based on the performance of the constituent species in monoculture. Antagonistic or negative interactions, such as interference competition for shared and limited resources, result in community growth being worse than the sum of the expected species yields. Additive mixing effects correspond to the sum of the expected monoculture yields (dashed line) and occur if species use non-overlapping resources and do not interact otherwise. Synergistic effects result in higher than expected biomass production and occur if one or both species benefit in mixtures due to complementarity or facilitation. (Modified from Fiegna et al. 2015)
Mathematical approaches are helpful to detect complementarity effects and to distinguish them from selection effects (Box 20.5), but they do not indicate a specific biological process responsible for the observed effects. Thus, other approaches are needed to obtain a more mechanistic understanding of the role of plant diversity in ecosystems. For instance, below-ground resource complementarity can be quantified by determining the species-specific root distribution within the soil or by injecting nutrient or water tracers (e.g. distinct isotopes of nitrogen or water) at different soil depths, at different times of the year or in different chemical forms and measuring their uptake by plants. Above-ground, niche differentiation and complementarity for light can be related to the surface of light-absorbing tissues (mainly LAI) and community light capture (light attenuation within the canopy) or to detailed measures of canopy packing or space filling. Values of the LAI and other measures of plant canopy complexity often increase with increasing diversity, as well as with densities of the vertical stand profiles and community heights, all being indicative of higher canopy packing. Optimised canopy packing, which fills the available space more completely with photosynthetic active tissue in both space and time (e.g. through phenological niche differentiation), can thus be an important mechanism of above-ground resource complementarity to increase total light interception and canopy photosynthesis.

Potential tree species richness effects on canopy packing. a 3D illustration of canopy packing within a 30 × 30 m plot, with maximum tree height (Hmax), the lowest crown base (defined as the difference between tree height (H) and crown depth (CD) (H-CD)min) and the volume of individual tree crowns (blue and red shapes). The grey shaded region depicts the potentially available space to grow in. b Canopy packing can be increased by mixing species that occupy different vertical strata. c Alternatively, canopy packing can also be optimised by plastic expansion of crowns to reduce competition with neighbours (Jucker et al. 2015). Reproduced with permission from John Wiley & Sons
For example, using a network of 209 permanent forest plots across Europe, spreading a gradient of tree species richness at each of six sites, Jucker et al. (2015) were able to show that canopy packing increased significantly with the number of tree species within a stand. This positive diversity–canopy packing relationship was partly driven by differences in vertical crown stratification between different species (i.e. by mixing species with different canopy heights) (Fig. 20.40b). In addition, species increased crown volume (i.e. developing longer lateral branches and deeper crowns) in mixture compared to monocultures (+38%), thereby showing phenotypic plasticity in response to hetero-specific neighbours (Fig. 20.40c).
Increased canopy packing by mixing species will enhance light interception, may increase litter production and alter microclimatic conditions and offers higher structural complexity. These changes will further affect productivity, biogeochemical fluxes and the diversity of other organisms. Thus, managing forests with the goal of safeguarding these functions may be achieved by increasing canopy packing and its structural complexity through mixing of tree species. However, there might be trade-offs between different management goals. For example, increased canopy packing may offer more structural heterogeneity and, thus, more habitats for canopy-living organisms, such as insects or birds (Fig. 20.6). But it may also result in higher light absorption in the tree canopy, and thus lower light availability at the forest floor for understorey vegetation, as well as cooler and dryer soil conditions that also negatively affect nutrient cycling. This highlights the need for local, site-specific management decisions, which might include a weighted valuation of different ecosystem functions or services (e.g. higher value for birds than for understorey plants) or which might include additional silvicultural interventions (e.g. reducing stand density to allow greater light penetration to the understorey).
20.4.9.3 Facilitation and Mutualism
Plants living in close proximity to each other commonly have demands for the same resources and therefore compete with each other. However, plants may also benefit from their neighbours through the amelioration of the physical and biotic environment. If such positive interactions among different species enhance the availability and, hence, capture of resources, then this can also generate a positive relationship between biodiversity and ecosystem processes. For example, symbiotic fixation of atmospheric nitrogen by some plant groups, such as legumes, increases the overall availability of nitrogen to the whole community through decomposition of the legume residues rich in N and through reduced competition for soil N (Sects. 7.4 and 16.3). Thus, more biomass can be produced than in the absence of legumes. This N-fertilisation effect is commonly applied in managed grasslands and in intercropping to increase yields, for example, by mixing grasses with clover species or corn with beans, respectively. Hence, positive plant diversity effects on productivity are observed not only in natural or low-intensity grasslands but also in intensively managed grass–clover leys (e.g. Kirwan et al. 2007). Hydraulic redistribution or lift is another example of facilitation, where deep-rooting species (e.g. trees) take up water from deeper soil layers and release some parts of that water through their roots in upper, drier layers. This water can then be taken up by other, more shallow-rooting species, such as grasses, which therefore benefit from the presence of the trees (Sect. 10.2). Because it is difficult to distinguish facilitative or mutualistic interactions from niche differentiation—in fact, there are many transitions between them—both aspects are often included in a general concept of complementarity.
20.4.9.4 Trophic Interactions and Negative Density -Dependent Effects
Negative density-dependent effects mediated by other trophic levels, in particular seed predators, pathogens and herbivores, may also lead to a positive diversity–productivity relation. Janzen (1970) and Connell (1971) proposed that specialised seed predators would lead to a negative feedback on tree population growth that depends on the density and distance distribution of host trees (Sect. 19.3). While such Janzen–Connell effects have been proposed as an explanation of the high diversity of coexisting trees, they could also explain higher productivity in species-rich communities. In fact, the proportionally low productivity in plant monocultures compared to mixtures can be increased by (partial) soil sterilisation, which destroys fungi, bacteria, nematodes and other pathogenic organisms. These host-specific pathogens and herbivores induce large losses in plant biomass or cause more photosynthates to be diverted to defence mechanisms, leaving less carbon for growth (Sects. 8.1–8.3). Thus, the accumulation of host-specific pathogens over time produces strong negative effects, especially in monocultures or low-diversity mixtures owing to the large abundance of the host species, which facilitates the fast spread of pathogens. This effect have been known for a long time to farmers (“soil fatigue”), who avoid planting the same crop species in consecutive years. Yearly crop rotations are a means to avoid these negative plant–soil feedback effects. In mixed communities, such negative feedback will be diluted owing to the lower abundance of specific host species, thereby promoting a positive biodiversity–biomass relationship (van der Putten et al. 2013). In addition, there might also be facilitative , beneficial soil organisms for plants (e.g. microbes in the rhizosphere enhancing mineralisation rates, N-fixing bacteria, mycorrhizal species) (Sects. 7.4 and 19.4) that could accumulate over time, an effect that would be stronger in plant mixtures because different plant species attract different mutualists. Over longer stretches of time, the stronger effects of negative plant–soil feedbacks in monocultures, together with positive interactions that lead to an increasing per capita performance in mixtures, contribute to the observation that the slope of the plant diversity–ecosystem functioning relationship often becomes steeper over time (Eisenhauer et al. 2012).
20.4.10 Value of Biodiversity–Ecosystem Functioning Research
After almost two decades of intensive research, biodiversity–ecosystem functioning science has come into a phase of synthesis, fostered by several reviews (Kinzig et al. 2002; Loreau et al. 2002; Naeem et al. 2009; Weisser and Siemann 2004; Scherer-Lorenzen et al. 2005; Hooper et al. 2005; Tilman et al. 2014) and meta-analyses (Balvanera et al. 2006; Cardinale et al. 2006, 2007, 2011). The insights gained are all highly relevant for our fundamental understanding of how ecosystems work and how plant diversity controls ecosystem processes. However, how reliable are results from biodiversity–ecosystem functioning research, which is still largely based on some “artificial” experimental manipulation? Are biodiversity experiments suitable to predict the consequences of species changes in real ecosystems? Can the results from biodiversity–ecosystem functioning studies guide us in developing effective nature conservation or ecosystem management strategies? Can we design sustainable production systems based on the insights obtained from knowledge of biodiversity–ecosystem functioning? Especially for experimental approaches, these questions have generated controversy, despite the fact that they focus on general patterns and ecological mechanisms and less on applied problems of nature conservation (for an overview, see Duffy 2009).
20.4.10.1 Critical Views on Biodiversity–Ecosystem Functioning Experiments
The effects of plant diversity on ecosystem functions often reach an asymptote at rather low levels of diversity in experiments, suggesting redundancy among species at higher diversity levels, while natural ecosystems are often composed of many more species at similar spatial scales. This is only true for short-term experiments, and redundancy has been shown to decrease with the duration of experiments. Redundancy also mostly applies to single functions and is less plausible for multiple functions (Fig. 20.33). In addition, several studies that compared ecosystem functions across gradients in plant diversity in natural ecosystems also found similar diversity effects (e.g. Fig. 20.26). Finally, well-documented species–area relationships (Sect. 18.4) suggest that maintaining a given level of diversity at the local scale requires the conservation of species at regional scales.
Diversity effects are often a result of statistical sampling or selection effects (Sect. 20.4.9), which, unlike resource complementarity, has not been considered to be a “true” diversity effect (Huston 1997). However, sampling or selection effects mostly fade out with the duration of experiments, with complementarity becoming stronger over time. The sampling effect also focuses on single response variables. The maintenance of a variety of ecosystem functions and services provided by real, complex ecosystems requires a large number of species because there is no “super-species” that could provide such different functions at high rates and in several different contexts (Fig. 20.33).
Biodiversity experiments usually involve a substantial effort to maintain the gradient in species richness, for example, by weeding out colonising species, which usually is higher at low than at high diversity. They also often include species that are unlikely to occur in monoculture under the environmental conditions of the site or include species combinations rarely found growing together in nature. These aspects can result in rather low cover of the target species in monoculture or low-diversity mixtures, which would decrease the mean rates of ecosystem functions, such as biomass production, and hence introduce some potential artefacts in biodiversity–ecosystem function relationships. Several studies have now shown that weeding is actually not as detrimental as suspected and that the poor performance of species in monoculture is rather the result of an attack by various pathogens. Thus, higher weeding effort in monocultures could be largely the consequence—and not the cause—of poor monoculture performance.
One major critique is related to the predominant use of random extinction scenarios to create a gradient of plant diversity in experiments, while species loss in real ecosystems is usually not random but is based on species abundance, rarity and vulnerability. One might postulate that loss of less abundant, rare species should have fewer effects than a random scenario because the remaining and dominant species could compensate for the loss. However, theoretical studies, simulations and experiments that applied non-random extinction scenarios also found diversity effects on several ecosystem processes, sometimes even stronger than those based on random-loss designs. One could also argue that applying a random-loss order is a good starting point to study the consequences of diversity changes because future causes of species extinctions might be different than current ones. In fact, studying the effects of diversity change in experiments has produced an astonishing wealth of knowledge about the general nature of the relationship between biodiversity and ecosystem functioning (Schmid and Hector 2004). Nevertheless, the scientific evidence for consequences of realistic, non-random extinction scenarios is still much weaker than that of random scenarios, leaving room for further investigations.
Confusion often arises owing to the fact that plant diversity often declines as productivity increases at high levels of resource supply (“paradox of enrichment ”) (Sect. 20.3.3), while the “opposite” is mostly found in studies examining the ecosystem effects of changing diversity (Sect. 20.4.2). However, these views are not contradictory but rather focus on two different angles on the bidirectional relationship between species diversity and productivity : the former illustrates the response of species diversity to changing resource availability across ecosystems, which determines the potential for biomass production and diversity. In contrast, biodiversity experiments study the consequences of diversity changes for productivity (and other functions) within ecosystems in which resource levels are held constant. Thus, at any given level along a resource gradient, the availability of resources will determine the number and composition of species, while a reduction of species at this resource level will mostly result in reduced biomass production.
20.4.10.2 Relevance of Biodiversity–Ecosystem Function Research for Sustainable Production Systems, Nature Conservation and Ecosystem Management

Conceptual graph showing the potential of using high crop diversity to achieve high yields. The upper solid curve shows the diversity–yield relationship under conditions of low environmental variability (e.g. constrained by management interventions). The lower dashed line shows the same relationship under conditions of large spatial or temporal environmental heterogeneity (e.g. where it is too costly or impossible to homogenise the environment). Blue dots represent single, very high productive species. The insets show yield responses of single species along environmental gradients, and the blue shaded region represents the range of environmental conditions exhibited overs space and time (Modified from Isbell et al. 2017)
Finally, it can be questioned whether changes in biodiversity can have effects comparable to those of other drivers of global change , such as eutrophication , climate change or land-use change , which have significant impacts on ecosystems (Chap. 23). At least for biomass production, there is accumulating evidence that the effects of species loss are on the same order of magnitude as those of eutrophication in the form of nitrogen deposition or nutrient fertilisation, drought stress, fire, herbivory or elevated CO2 (Hooper et al. 2012; Tilman et al. 2012). Thus, the global loss of biodiversity we are facing today is certainly one of the major drivers of changes in ecosystem functioning and, thus, is also strongly affecting the delivery of ecosystem services for humanity. Hence, besides ethical considerations, the strong control that biodiversity has on ecosystem multifunctionality and stability should be another, utilitarian argument for conservation and restoration of biological diversity in the era of human dominance of almost all global ecosystems.
Nevertheless, we close this chapter with a note of caution: high biodiversity or high levels of ecosystem functioning are often a reasonable goal for nature conservation or ecosystem management , but they are not always “good” or “desired”: for example, an increase of plant diversity in naturally species-poor ecosystems, such as raised bogs, is often indicative of substantial changes in site conditions, for example, through drainage. Similarly, an increase in productivity in oligotrophic ecosystems, such as acidic grasslands, is also often due to human-induced changes in resource availability, for example, through fertilisation or atmospheric nitrogen deposition. We should therefore base conservation efforts not only on general biodiversity–ecosystem functioning relationships, but on ecosystem- and site-specific targets of biodiversity (e.g. rare species, keystone and umbrella species, representative communities, natural seral communities) and ecological processes (e.g. predator–prey interactions, pollination, soil stabilisation, carbon sequestration).
20.5 Summary
-
Biodiversity—or biological diversity—encompasses the variability of biological entities from all sources, including, inter alia, terrestrial, marine and other aquatic ecosystems, and the ecological complexes of which they are part, across all levels of biological hierarchies. This includes diversity within and between species and of ecosystems.
-
Biodiversity can be categorised into compositional, structural and functional diversity, focusing on the elements that account for biodiversity and the resulting physical structures and functional consequences for ecosystems.
-
Plant (functional) traits encompass morphological, anatomical, biochemical, physiological and phenological properties of organisms that reflect their evolutionary history and shape their performance. They have a direct or indirect impact on the fitness of plant individuals through their effects on growth, reproduction and survival. Traits strongly determine the ecological role of plants within a community through their responses to environmental factors and their effects on other trophic levels or ecosystem processes.
-
Plant species have evolved in certain areas and are adapted to specific environmental conditions. Hence, plant diversity shows striking spatial patterns at various spatial scales, reflecting the role of environmental filters that control species presence and absence. The availability of energy and water and environmental heterogeneity and disturbance regimes are major factors affecting diversity patterns. Human land use and management has strongly modified these patterns.
-
Plant species richness sometimes peaks at intermediate levels along gradients of site productivity (humped-back productivity–diversity model). However, both productivity and richness are influenced by a multitude of factors and processes, making multivariate controls of plant diversity more relevant than simple bivariate relationships.
-
Loss of biodiversity and alterations in plant species composition can influence ecological processes and functions within ecosystems, resulting in “biodiversity–ecosystem functioning” relationships. These can be driven either by the degree of dominance (i.e. abundance) and the identity (i.e. presence and value of functional traits) of the dominant species or by antagonistic or synergistic interactions among co-occurring species.
-
There is often a positive relationship between plant diversity and multiple ecosystem processes. More diverse systems consistently have higher biomass production, higher nutrient uptake and, consequently, lower leaching losses to the groundwater, and they are more resistant to pathogens or invasion by other species than the corresponding monocultures. However, polycultures often produce less biomass than the highest yielding species grown in monoculture and retain lower amounts of soil resources than the most efficient monoculture. Loss of biodiversity usually increases temporal stability and the resistance of ecosystems in the face of disturbances.
-
Mechanisms underlying the observed biodiversity–ecosystem functioning relationship include sampling and selection effects, resource complementarity among species due to niche differentiation, facilitation and mutualisms, or trophic interactions.
-
Biodiversity–ecosystem functioning research has greatly improved our general understanding of community and ecosystem ecology, but it also has consequences for ecosystem management and the design of sustainable production systems in agriculture or forestry.